Ebb-and-Flow Bioreactor
Regime and Electrical
Elicitation: Novel
Strategies for Hairy Root
Biochemical Production
Joel L. Cuello and Lisa C. Yue
The University of
Arizona, Department of Agricultural and Biosystems Engineering,
507 Shantz Building, Tucson, AZ
*Corresponding author; email:
cuelloj@mail.arizona.edu
Keywords:
ebb-and-flow bioreactor, hairy roots, electrical elicitation
ABSTRACT
The ebb-and-flow
bioreactor regime and electrical elicitation constitute two
novel and promising effective strategies for realizing the
large-scale production of secondary metabolites from hairy roots
growing in scaled-up bioreactors. Employing the ebb-and-flow
regime would provide the forced-convective action that is needed
to overcome the mass-transfer resistances offered by the
stagnant external boundary layers and/or dead zones which
normally exist in the high-density hairy-root bioreactor
environment – both in liquid-phase and in gas-phase bioreactors.
Meanwhile, employing electrical elicitation could significantly
overproduce valuable secondary metabolites produced by hairy
roots. Combining the two strategies could certainly help achieve
the economic feasibility of the large-scale production from
plant hairy roots of specific secondary metabolites.
INTRODUCTION
Plant roots have long been known as prolific manufacturers of an
assortment of economically important chemicals. Since Dawson
(1942) first showed conclusively that the roots of Nicotiana
tabacum were the site of synthesis of the secondary
metabolite nicotine, numerous root cultures representing a wide
range of species have been demonstrated to produce diverse kinds
of phytochemicals in vitro. The advent of transgenic
hairy roots has presented a novel and versatile system for
production of plant chemicals (Kamada et al., 1986; Flores et
al., 1993; Mahagamasekera and Doran 1998; Medina-Bolivar et al.,
2007) and even of transgenic proteins (Medina-Bolivar et al.,
2003; Medina-Bolivar and Cramer 2004). Hairy roots have a
typical doubling time in culture of 2 to 4 days, grow
significantly faster than untransformed roots, are stable in
culture, grow significantly faster than most suspension cell
cultures, and generally produce the same if not higher levels of
metabolites as do normal roots and cell suspension cultures
(Hamill et al., 1987; Flores et al., 1987; Kwok and Doran 1995).
This paper describes the ebb-and-flow bioreactor regime as a
novel and promising effective strategy for overcoming the
scale-up challenges that stand in the way of realizing the
large-scale production of desired phytochemicals from
immobilized hairy root cultures growing in bioreactors. This
work also describes electrical elicitation as a novel and
promising effective strategy for significantly increasing the
production of secondary metabolites by hairy roots in
large-scale bioreactors.
EBB-AND-FLOW BIOREACTOR
REGIME
Hairy Root Bioreactor
Challenges
The mass production of chemicals derived from hairy roots
requires the development of scaleable hairy root bioreactors.
However, the tangled and fibrous-clump morphology which the
hairy roots assume under high-density conditions in bioreactors
which is conducive to creating external boundary layers over
root surfaces and around the root clumps and stagnant zones
within the root clumps presents significant mixing and
mass-transfer challenges for liquid-based delivery of nutrients
and oxygen to the roots. Oxygen's low solubility in aqueous
medium and its poor mass transfer through the medium into the
root cells simply make O2 the most critical limiting
factor in hairy root bioreactor design (Yu and Doran 1994;
Ramakrishnan and Curtis 1995; Kanokwaree and Doran 1997;
Tescione et al., 1997).
In general, a hairy root bioreactor has to fulfill four minimum
requirements upon scale up (Cuello 1994): (1) its characteristic
configuration (e.g., flow configuration, geometric
configuration, etc.) must remain operative, and not degrade,
upon scale up; (2) it should make the nutrients and oxygen
available, or accessible, to the roots throughout the reaction
volume; (3) it should supply both nutrients and oxygen to the
roots in sufficient concentrations throughout the reaction
volume; and (4) it should lend itself to the scale up process by
possessing identifiable and quantifiable characteristic process
parameters which can be duplicated at larger scales with
relative ease and economy.
Various configurations for small-scale hairy root bioreactors
have been employed since the mid 1980’s. Rhodes et al., (1986)
were the first to demonstrate the successful growth of a hairy
root culture in a bioreactor for pharmaceutical production, and
were also first to employ a submerged convective-flow
configuration in a root bioreactor, growing Nicotiana rustica
hairy root culture in an 880 mL continuous convective flow
bioreactor (CFR). Seminal studies on other types of hairy root
bioreactor configuration were conducted by: (1) Taya et al.,
(1989) for bubble column, immobilized bubble column, immobilized
trickle-bed column and immobilized fill-drain (ebb-and-flow)
column bioreactors; (2) Kondo et al., (1989) for turbine-blade,
rotating-drum and immobilized rotating-drum bioreactors; (3)
Hilton and Rhodes (1990) for modified batch and continuous
stirred-tank bioreactors; and, (4) DiIorio et al., (1992) for a
nutrient mist bioreactor. Cuello et al., (1991) were the first
to demonstrate the successful growth of a hairy root culture in
an ebb-and-flow bioreactor. Subsequent studies on hairy root
bioreactors were reviewed by Singh and Curtis (1994) and Choi et
al., (2006), among others.
Of the foregoing hairy root bioreactor configurations, only a
few can be expected in theory to sustain the successful growth
of high-density hairy root cultures upon scale up to
significantly larger volumes. For a simple bubble-column design,
the dense packing of root clumps would promote bubble coalescing
and channeling, leading to poor liquid mixing, localized liquid
stagnation and, ultimately, to severe oxygen and nutrient
maldistributions and limitations. A fine-mist configuration
would be expected to break down into a trickle-bed configuration
along the length of the bioreactor, since fine mists would not
remain as such after they enter into the dense root bed (Flores
and Curtis 1991). Although the trickle-bed configuration appears
to be scaleable, it has the potential problem of being
susceptible to liquid channeling and liquid stagnation. A
stirred-tank bioreactor, with the roots immobilized and securely
removed from the impeller zone, would likely have problems of
liquid stagnation along the reactor periphery. Meanwhile, a
horizontal rotating drum bioreactor appears to have scale-up
potential. With the drum partially filled with liquid medium,
its immobilized roots would be exposed to alternating gas and
liquid phases. This design, however, is complicated by the
gradient in linear velocities experienced by the roots along the
radius of the reactor.
The continuous CFR and the ebb-and-flow bioreactor (EFBR), both
exhibiting saturated tubular convective flow part of the time in
the case of the EFBR possess the advantage of liquid and gas
mixing through the root matrix, which can be maintained upon
scale up. Being continuous systems (with inflow and outflow),
both are also ideal configurations for the following scenarios:
(1) when the roots require separate biomass accumulation stage
and secondary-metabolite production stage, requiring a change in
nutrient medium during the course of the growth period; (2) when
the concentration of dissolved oxygen in the liquid medium needs
to be brought back periodically to a certain level, requiring
re-aeration of the liquid medium in a separate well-mixed
reservoir; and (3) when the extracellular secondary metabolites
excreted by the roots into the liquid medium need to be
continually extracted from the liquid medium through in situ
product removal (ISPR) to prevent feedback inhibition. Both the
CFR and EFBR, however, would be expected to have critical limits
in their heights to prevent the dissolved-oxygen concentrations
in the bulk liquid from becoming growth-limiting to the roots.
The EFBR has the following three principal advantages over the
CFR: (1) expected better mixing and mass-transfer capacities
owing to the recurrent back-and-forth liquid flows and
intermittent liquid and gas phases; (2)
intermittent exposures to gas phase, allowing for more
effective manipulation of the gas composition (e.g., possible
addition or removal of certain gases such as oxygen, carbon
dioxide or ethylene) for further optimization of root growth or
phytochemical production; and (3) the ability to alleviate the
significant energy penalty imposed by the high pressure drop
created by the dense root bed by simply flooding the roots with
the nutrient medium during the Fill Time (i.e., during the flow
phase of the ebb-and-flow regime) and letting the nutrient
medium drain out of the root bed by gravity or as assisted by a
pump during the Drain Time (i.e., during the ebb phase of the
ebb-and-flow regime). In contrast, a CFR requires that its
liquid nutrient medium be pushed in bulk through the root bed
continuously, incurring the full energy penalty of the root
bed’s high pressure drop during the entire bioreactor run.
The Ebb-and-Flow
Bioreactor
The ebb-and-flow bioreactor derives its name from the process
behavior of its liquid medium which is characterized by its
repetitive ebbing and flowing or periodic filling and draining
(Figure 1). The reactor may be considered as the common-sense
compromise between the two extreme bioreactor configurations of
the predominantly liquid-phase and the predominantly gas-phase.
The ebb-and-flow bioreactor has four characteristic operational
phases which recur sequentially and intermittently as the liquid
medium moves back and forth between the bioreactor vessel and
its reservoir (Figure 2). These include the liquid dwell time
(LDT), the drain time (DT), the gas dwell time (GDT) and the
fill time (FT). The LDT is the phase where the whole reaction
volume of the EFBR is completely submerged in liquid, and where
the bulk of the liquid medium is neither flowing upward nor
downward. The GDT is that operational phase where the EFBR
reaction volume is predominantly in the gas phase, and where
mass flow of the bulk liquid medium is not occurring. The
operational phases where the bulk flow of the liquid medium
takes place are the FT, when the bulk flow direction is upward,
and the DT, when the bulk flow direction is downward.
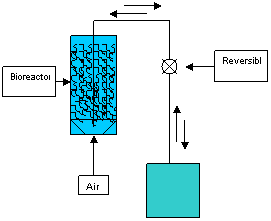
Figure 1.
Schematic of an ebb-and-flow bioreactor, showing the bioreactor
vessel containing the hairy roots and a programmable reversible
pump that moves the liquid nutrient solution back-and-forth
between the bioreactor vessel and the reservoir. Compressed air
is sparged through the bottom of the bioreactor vessel.
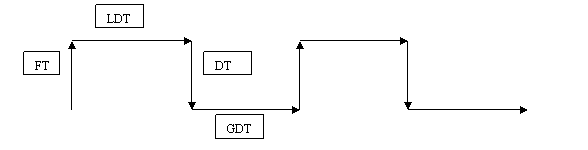
Figure 2.
The four characteristic operational phases of the ebb-and-flow
bioreactor which recur sequentially and intermittently as the
liquid medium moves back and forth between the bioreactor vessel
and its reservoir. FT = fill time, LDT = liquid dwell time, DT =
drain time and GDT = gas dwell time. Both x and y axes are time
scales. The direction of the arrows pertains to process flow.
Ebb-and-Flow Bioreactor Vs. Bubble Column
Cuello et al. (2003a, 2003b) compared the growth performance of
a 2.5-L EFBR (Figure 3) against that of a bubble column, also
called a submerged sparged bioreactor (SSBR). The EFBR was
operated with the following values of operational parameters:
LDT = 2 min; GDT = 2 min; FT = 1 min; and DT = 1 min. It was
operated in two stages: first, under a bubble column regime for
the first seven days of growth; and, second, under the
fill-and-drain process for the succeeding time period. The
normal EFBR treatment was sparged at an air flow rate of 2.72
mL/s, equivalent to 0.115 vvm, during the entire growth
duration. At the start of the reactor run, the EFBR vessel
contained 2000 mL of liquid medium while the reservoir contained
500 mL. Note that the working volume inside the bioreactor
vessel was only 2000 mL.
Three types of controls were used in discriminating the effects
of the ebb-and-flow process. These controls included: (1) the
Erlenmeyer shake flasks; (2) a recirculating SSBR; and (3) a
nonrecirculating SSBR. The shake flasks used were of two sizes:
the 125 mL size and the 1000 mL size containing 50 mL and 250 mL
of liquid medium, respectively. The rationale for using a
recirculating SSBR control was to keep the total volume of the
liquid medium for this control the same as that for the EFBR
treatment which was 2500 mL. Similar to the EFBR, the
recirculating SSBR contained 2000 mL of liquid medium in the
bioreactor vessel and 500 mL in its reservoir at the beginning
of a reactor run. Operated as a bubble column with the same
sparging rate as the EFBR for the first seven days, the
recirculating SSBR was then operated starting on the eighth day
so that its liquid medium was recirculated between its cylinder
and reservoir at a rate averaging 0.5 mL s-1, a flow
rate that was significantly lower than that of the EFBR. During
the FT and DT operational phases of the EFBR, the flow reached
its minimum value at an average of 14.4 mL s-1 on day
18. The third control, a nonrecirculating SSBR which contained
only 2000 mL of liquid medium in its cylinder (it had no
reservoir), was somewhat of a control for the recirculating SSBR
to demonstrate that any effects of the low convective flow
produced by the recirculation of liquid medium were negligible.
The 125 mL flask control was referred to as 50 mL flask control,
the 1000 mL flask as 250 mL flask control, the recirculating
SSBR as SSBR, and the nonrecirculating SSBR as SSBRnonrec.
The results by Cuello et al., (2003b) clearly demonstrated the
EFBR’s successful scale up of the 50 mL flask to a 50X scale.
The 2.5 L EFBR produced an average dry weight of 21.64 g in 18 d
(Figure 3) which was statistically indistinguishable from the
combined average dry weights after 18 d of fifty 50 mL shake
flasks of 23 g, at
a = 0.05, and of ten
250 mL shake flasks of 20.3 g, also at
a
= 0.05. Note that these comparisons were based on an equal total
volume of the liquid medium, 2500 mL. By contrast, the combined
average dry weights for the 250-mL flasks significantly fell
short (a
= 0.05) of those for the 50 mL flasks, showing the failure of
the Erlenmeyer flask to be amenable to the scale-up process.
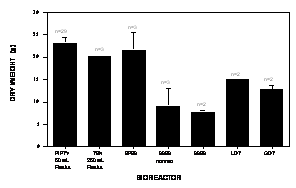
Figure 3.
Growth comparison of Hyoscyamus muticus hairy root
cultures after 18 days in bioreactors with 2500 mL of B5 medium;
n = number of observations. EFBR = ebb-and-flow bioreactor; SSBR
= submerged sparged bioreactor; LDT = EFBR with LDT of 5 min;
GDT = EFBR with GDT of 8 min.
Comparing the EFBR against the SSBR, the EFBR's average dry
weight significantly exceeded by 138% (a = 0.05) that for
the SSBR of 9.08 g. It was noteworthy that there was no
significant difference (a
= 0.05) between the average dry weight of 7.56 g for the SSBRnonrec
and that for the SSBR, indicating that the SSBR indeed had
negligible convective flow and that it was the back-and-forth
convective flow of the EFBR that made it superior to the SSBR.
It also showed that air bubbling, which was present in both EFBR
and SSBR, was by itself insufficient to maintain root growth at
larger scale.
The bioreactors' average specific growth rates (Table 1)
reflected the same trends exhibited by their dry weights. No
significant statistical differences existed between the average
specific growth rates of the EFBR (0.211 d-1) and the
two shake-flask controls (0.218 d-1 for 50 mL flask
and 0.217 d-1 for 250 mL flask, both at
a
= 0.05), but the average specific growth rate of the EFBR
significantly exceeded by 41% (a
= 0.04) that of the SSBR (0.150 d-1). Also, the
average specific growth rate of the SSBR was not
significantly different (a
= 0.05) from that of the SSBRnonrec (0.142 d-1).
Table 1.
Comparison of average specific growth rates (μ) of Hyoscyamus
muticus hairy root cultures grown in different bioreactor
treatments for 18 days; n = number of observations. EFBR =
ebb-and-flow bioreactor; SSBR = submerged sparged bioreactor;
LDT = EFBR with LDT of 5 min; GDT = EFBR with GDT of 8 min.
Treatment |
(day-1) |
n |
50 mL flask |
0.219 ± 0.004 |
29 |
250 mL flask |
0.217 ± 0.0000 |
3 |
EFBR |
0.211 ± 0.016 |
3 |
SSBR |
0.150 ± 0.026 |
3 |
SSBRnonrec |
0.142 ± 0.001 |
2 |
LDT |
0.188 ± 0.001 |
2 |
GDT |
0.181 ± 0.004 |
2 |
Cuello et al., (2003b) found that the EFBR and the SSBR growth
profiles over time did not exhibit a marked divergence in their
daily dry weights until after day 14. The subsequent rapid
increase in the dry weight of the EFBR distinctly contrasted
with the smaller growth increments exhibited by the SSBR. This
result was supported by visual observations. No appreciable
differences were observed between the roots growing in the EFBR
and those in the SSBR controls until after the first 10 d of
their growth period. It was then when the roots in the EFBR as
well as in the SSBR controls started developing into discrete
root clumps, which continued to grow and expand and, by day 14,
eventually interlocked with neighboring root masses to form a
tangled, porous matrix of fibrous hairy roots. At this time,
significant coalescing and channeling of the sparged air bubbles
during the liquid-phase duration were observed, suggesting the
formation of localized stagnant regions within the root clumps
as well as the formation of significant external boundary
layers. It was also at this time when the difference between the
EFBR and the SSBR controls in terms of both growth rate and
accumulated biomass sharpened significantly.
Comparing the SSBR and the EFBR hold-up profiles upon drainage
(Figure 4), Cuello et al., (2003b) found that the residual
hold-up for the SSBR progressively declined after successive
draining of its liquid medium. By contrast, the residual hold-up
for the EFBR after successive draining remained essentially
constant. While the EFBR consistently held up approximately the
same volume of medium after each time that the bulk liquid was
drained, the SSBR exhibited time-dependency in its residual hold
up, with diminishing hold-up levels upon drainage repetition.
After repeated filling and draining, the residual hold-up of the
SSBR continually decreased and approached that of the EFBR.
These results indicated that, in comparison with the EFBR, the
SSBR possessed significantly more external boundary layers
around the roots and/or stagnant zones that provided significant
resistance to mass transfer of oxygen and nutrients. Meanwhile,
the bi-directionality of the forced convective flow in the EFBR
and the repetitive filling and draining of its bulk medium
successfully ensured the periodic attenuation of both the
boundary layers and any stagnant zones within the EFBR. The
sugar profiles for the EFBR and the SSBR showed that, on day 18,
the total sugar level in the EFBR was 8.9 g L-1, a
level that was considerably lower than that in the SSBR of 14.0
g L-1 for the same day. This result confirmed that
the SSBR control, relative to the EFBR, was hampered by sugar
mass-transfer limitation. And given the much lower solubility of
oxygen in the liquid medium, and the finding that the oxygen
consumption rate in the EFBR and SSBR remained statistically the
same at an average value of 0.05 mg min-1 g-1
DW, it could safely be inferred that the SSBR was also hampered
by oxygen mass-transfer limitation. The foregoing results on the
poor growth performance of the SSBR and the accompanying
observations about its hydrodynamic behavior are consistent with
those reported by others (Shia and Doran 2000; Kanokwaree and
Doran 2000).
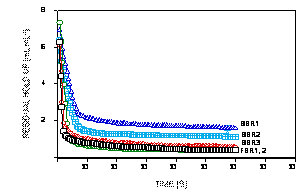
Figure 4.
Time profiles of residual hold-up for normal ebb-and-flow
bioreactor (EFBR) treatment and submerged sparged bioreactor
(SSBR) growing Hyoscyamus muticus hairy roots at tissue
concentrations of 267 and 283 g L-1 FW, respectively.
Profiles obtained on successive drainage are designated by 1,
2, etc., in chronological order.
EFBR with Prolonged Liquid
Phase and EFBR with Prolonged Gas Phase
Cuello et al., (2003b) also ran an EFBR treatment with a
prolonged liquid phase, called LDT treatment, whose LDT = 5 min,
GDT = 2 min, FT = 1 min and DT = 1 min. Likewise, an EFBR
treatment with a prolonged gas phase, called GDT treatment,
whose GDT = 8 min, LDT = 2 min, FT = 1 min and DT = 1 min, was
also performed.
Their results (Figure 3) showed that the LDT treatment's average
dry weight of 14.99 g and the GDT treatment's average dry weight
of 12.74 g fell between those of the EFBR and the SSBR control,
that is, below that of the EFBR and above that of the SSBR
control. Although the average dry weights of the LDT and GDT
treatments remained statistically indistinguishable from that of
the normal EFBR treatment, both at
a
= 0.05, the average dry weights of the LDT and the GDT
treatments were nonetheless 31% and 41% lower than that of the
normal EFBR treatment, respectively. Indeed the average dry
weights of the LDT and the GDT treatments became significantly
lower than that of the normal EFBR treatment at
a
= 0.12 and
a
= 0.06, respectively. Equally noteworthy, the average dry
weights of both the LDT and the GDT treatments were
significantly lower (a
Ł 0.05) than those of
the 50 mL and the 250 mL shake-flask controls. At the opposite
lower end, though the average dry weights of the LDT and GDT
treatments remained statistically indistinguishable from that of
the SSBR, both at
a
= 0.05, the average dry weights of the LDT and the GDT
treatments nonetheless exceeded that of the SSBR by 65% and 40%,
respectively. These same trends were exhibited by the resulting
growth rates (Table 1).
The sugar profiles showed that, on day 18, the total sugar
levels in the LDT and GDT treatments hovered over 8.9 g L-1,
a level that was the same as that for the normal EFBR and again
considerably lower than that in the SSBR control of 14.0 g L-1
for the same day. These results showed that the sugars in the
GDT and LDT treatments were consumed to the same extent as that
in the normal EFBR treatment, indicating that, just as the
normal EFBR treatment did not suffer from sugar mass-transfer
limitation, neither the LDT treatment nor the GDT treatment also
suffered from sugar mass-transfer limitation. Otherwise, the
final sugar concentrations of the LDT and GDT treatments would
have been closer to the SSBR’s higher residual sugar
concentration of 14.0 g L-1.
Despite the LDT and the GDT treatments not experiencing
significant sugar mass-transfer limitations, however, the
dry-weight and growth-rate results (Figure 1 and Table 1)
indicated that the growth performances of both the LDT and GDT
treatments were on the verge of becoming significantly poorer
than that of the normal EFBR treatment. And, indeed, the average
dry weights of both the LDT and the GDT treatments were
significantly poorer than those of the 50 mL and the 250 mL
shake-flask controls (Figure 3). This was further supported by
the resulting maintenance coefficients for the bioreactors.
Recall that the maintenance coefficient of a root culture is the
portion of the total-sugar substrate it requires to maintain its
accumulated biomass. The maintenance coefficients of the LDT and
the GDT treatments of 1.09 and 1.49 g sugar g-1 DW,
respectively, were significantly greater than that of the normal
EFBR treatment of 0.30 g sugar g-1 DW and
statistically indistinguishable from that of the SSBR of 0.70 g
sugar g-1 DW, indicating that the roots in the LDT
and GDT treatments were expending via respiration a great
portion of their sugar substrates in maintaining their
accumulated biomass. The results demonstrate that the roots in
the LDT and GDT treatments were physiologically stressed, though
the cause of stress was clearly not sugar mass-transfer
limitations. Further, measurements of oxygen consumption rates
showed that the LDT treatment and the GDT treatment on day 18
also had an average value of 0.05 mg min-1 g-1
DW, which was statistically the same as that for the EFBR and
the SSBR.
Thus, the roots’ relative ability to consume oxygen could not
have accounted for the poor growth performance of the LDT and
the GDT treatments. All the bioreactors, however, were observed
to exhibit extensive bubble entrapment, coalescing and
channeling during their respective liquid phases, pointing to
the presence of external boundary layers as well as localized
stagnant regions within the bioreactors during the liquid dwell
time. Thus, without the benefit of more frequent or sufficient
forced convective flow generated by the ebb-and-flow action,
bioreactors with an extended or permanent liquid phase, like the
LDT treatment and the SSBR control, suffered from oxygen
mass-transfer limitations. The GDT treatment, also lacking a
sufficient forced convective flow to overcome the mass-transfer
resistances created by its stagnant root-entrained medium during
its prolonged gas phase, must have also experienced oxygen
mass-transfer limitation during the prolonged GDT. It was
noteworthy that the normal EFBR, the LDT treatment and the GDT
treatment all had the same root-entrained medium of 0.5 mL g-1
DW. Thus, even though the frequencies of forced convective flow
of 22.2% and 16.7% in the LDT and GDT treatments, respectively,
were sufficient to obviate overall sugar mass-transfer
limitations, such frequencies proved not enough to prevent
oxygen mass-transfer limitations in these treatments. Thus,
during the prolonged liquid phase of the EFBR, oxygen
mass-transfer limitation began to prevail, conforming to normal
expectation. During the prolonged gas phase of the EFBR,
however, oxygen mass-transfer limitation also began to prevail,
if counter-intuitively.
Implications for Scale Up
The study by Cuello et al., (2003b) reinforced that the forced
convective delivery of oxygen is the most critical variable for
the successful scale up of the EFBR and, indeed, of any hairy
root bioreactor. This finding agrees squarely with those from
previous studies on the critical necessity of liquid convective
flow on the effective delivery of dissolved oxygen to roots
grown in liquid medium (Prince et al., 1991; Yu and Doran 1994;
Singh and Curtis 1994; Shiao and Doran 2000; Ramkrishnan and
Curtis 2004). The study by Cuello et al., (2003b) also
underscored, however, the oxygen-delivery limitations that exist
in gas-phase bioreactors (also known as liquid-dispersed
bioreactors, including the nutrient mist, spray and trickle-bed
bioreactors) on account of the existence of stagnant external
boundary layers in these bioreactors in addition to the
potential occurrence of liquid channeling and water-logging
(Shiao and Doran 2000; Williams and Doran 2000; Ramkrishnan and
Curtis 2004). Quite simply, there is no convincing proof that
oxygen mass transfer limitation does not exist in these
gas-phase bioreactors. Weathers et al., (1999), for instance,
concluded from their study of 1.5 L nutrient-mist and
bubble-column bioreactors, based on mRNA levels of the
fermentation enzyme alcohol dehydrogenase (ADH), that the bubble
column was oxygen-limited since it exhibited expression of ADH,
while the mist bioreactor was not oxygen-limited since it did
not exhibit expression of ADH. (The bubble column yielded 1.7
times more dry biomass than the mist bioreactor.) Unfortunately,
however, the measurement of the activity of fermentation enzymes
such as ADH as an indicator of hypoxia in hairy root bioreactors
has proved unreliable, since cases have been reported where
oxygen-limited treatments and the non-oxygen-limited control
showed exactly the same levels of ADH activity, or the
oxygen-limited treatments failed to show elevated levels of ADH
activity (Shiao et al., 2002). Further, the treatments that
showed elevated levels of ADH activity ended up yielding greater
biomass growth, and the treatments that showed little or no ADH
activity ended up yielding lower biomass growth (Weathers et
al., 1999; Kim et al., 2002; Shiao et al., 2002). Thus, a
successful detection of ADH activity is neither a necessary nor
a sufficient indicator of the presence of oxygen limitation.
Conversely, failure to detect ADH activity is neither a
necessary nor a sufficient indicator of the absence of oxygen
limitation.
What is clear, however, is that there is a need both in
liquid-phase and in gas-phase bioreactors for a
forced-convective action to be employed in proper frequency to
overcome the mass-transfer resistances offered by the stagnant
external boundary layers and/or dead zones which normally exist
in the high-density hairy-root bioreactor environment. One way
to generate such forced-convective action is by employing the
ebb-and-flow regime, making the ebb-and-flow regime an effective
strategy for overcoming the scale-up challenges of hairy-root
production in bioreactors.
It is noteworthy that in compiling the results of 22 different
studies that presented 26 different hairy-root bioreactor
treatments (representing various combinations of different
bioreactor sizes, growth periods, and bioreactor types –
including stirred tank, bubble column, isolated impeller,
convective flow, nutrient mist, trickle/spray),
Ramakrish-nan and Curtis (2004) found that the bioreactor that
yielded the highest average specific growth rate -- for a
bioreactor that was at least 2 L in size, had a growth period of
at least 18 days, and had a dry biomass output of at least 10 g
L-1 was the ebb-and-flow bioreactor by Cuello (1994)
(Figure 5), which gave an average specific growth rate of 0.22 d-1.
It was telling that the one that came the closest to the EFBR
was a trickle-bed bioreactor by Ramakrishnan and Curtis (2004),
which gave an average specific growth rate of 0.21 d-1,
but one which was operated under an enriched-oxygen condition.
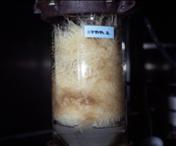
Figure 5.
A 2.5-L ebb-and-flow bioreactor (EFBR) during gas dwell time and
growing 18-day-old hairy roots.
It is worth pointing out that the performance of the normal EFBR
in the study by Cuello (1994) and Cuello et al., (2003b) had yet
to be optimized. With its FT and DT set constant at 1 min each
throughout the entire growth period while the hairy roots were
growing, the pump flow rate had to be continually reduced over
time so that it would deliver just enough liquid medium into the
bioreactor vessel to reach a constant level during each FT of 1
min. With more root biomass accumulating and occupying more
space inside the vessel over time, less and less liquid had to
be pumped into the vessel during FT. This meant that the flow
velocity of the nutrient medium through the root biomass in the
normal EFBR treatment kept being reduced just as the
mass-transfer resistance offered by the roots was increasing.
Clearly, there was room for further improvement of the
performance of the normal EFBR treatment.
Incidentally, the results by Cuello et al., (2003b) made clear
why the only previous attempt made by Taya et al., (1989) to
employ an ebb-and-flow bioreactor to grow immobilized hairy
roots (Armoracia rusticana) simply failed. Since their
300 mL EFBR used a liquid-phase duration of 15 minutes (i.e.,
much greater than the 4 min [FT + LDT + DT] used in the
foregoing study) plus a GDT of 8 h it could be safely inferred
that their system suffered from significant oxygen mass-transfer
limitations.
The EFBR can demonstrably meet all of the four minimum
requirements of a hairy root bioreactor for scale up. To wit:
(1) its characteristic configuration (e.g., flow configuration,
geometric configuration, etc.) remains operative, and does not
degrade, upon scale up; (2) it can make the nutrients and oxygen
available, or accessible, to the roots throughout the reaction
volume by proper adjustment of its LDT, GDT, FT and DT; (3) it
can supply both nutrients and oxygen to the roots in sufficient
concentrations throughout the reaction volume by proper
adjustment of its LDT, GDT, FT and DT; and (4) it lends itself
to the scale up process by possessing identifiable and
quantifiable characteristic process parameters which can be
duplicated at larger scales with relative ease and economy.
Examples of potential scale-up process parameters for the EFBR
include dissolved oxygen concentration at a specified location
in the bioreactor, volumetric flow rate, volumetric flow rate
per unit volume, Reynold’s number, power, and power per unit
volume, among others.
Finally, in scaling up the EFBR, there exists a maximum
allowable height for the EFBR column since the dissolved oxygen
in the liquid medium will be continuously consumed by the roots
as the liquid medium passes through the root bed along the
length of the EFBR column. It should be noted that the same is
true in the case of the continuous convective flow bioreactor
(CFR). Cuello (1994) recommended that, in industrial
applications, the most efficient and economical way of operating
the EFBR would be for two equal-sized EFBR’s to be operated in
tandem so that when one is drained, its medium is transferred to
fill the other. This mode of operation would obviate the need
for a costly sterile media reservoir which naturally would have
the same volume as that of the EFBR. Further, it was recommended
that LDT = GDT = 0 in order to maximize the allowable durations
for the FT and the DT. Indeed, their calculations showed,
adopting a value for the maximum tolerable flow velocity for the
roots of 16 cm s-1, that the allowable maximum height
for their specific EFBR design was 23 m (or 75 ft).This
corresponded to FT = DT = 3 min, specifying that that dissolved
oxygen was at saturation level in the liquid when introduced
into the EFBR at the beginning of FT and allowed to drop to no
less than 40% of saturation when the liquid exited the EFBR at
the end of the DT.
ELECTRICAL ELICITATION
Elicitation is the process whereby a molecule or a stress agent
induces a plant organ, tissue or cell to synthesize and
accumulate phytoalexins. Phytoalexins are antimicrobial chemical
compounds or secondary metabolites which in nature are
synthesized and accumulated by plants after exposure to
microorganisms (Darvill and Albershein 1984). Biotic elicitors
are molecules which are either pathogen-derived (so-called
exogenous elicitors, that is, with respect to the plant) or
plant-derived (so-called endogenous elicitors, also with respect
to the plant). Meanwhile, abiotic elicitors are physical or
chemical agents that induce physiological stress to plants,
leading to the synthesis and accumulation of phytoalexins.
Examples of abiotic elicitors include UV radiation, ethylene,
antibiotics, fingicides, salts of heavy metals, heat and cold,
etc.
The first biotic elicitor to be studied extensively, the peptide
monilicolin A, was obtained from the mycelia of the fruit-tree
fungal pathogen Monilinia fructicola (Darvill and
Albershein 1984). Oligosaccharides, polysaccaharides,
glycoproteins and organic acids derived from the mycelia and/or
cell walls of fungal microorganisms are some of the classes of
exogenous elicitors that have been reported, while
galacturonosyl residues have been reported to constitute a class
of endogenous elicitors (Darvill and Albershein 1984).
Examples of biotic elicitors that have been applied to hairy
root cultures to elicit the production of secondary metabolites
include those derived from Aspergillus niger for
Tagetes patula hairy roots (Buitelaar et al., 1993), those
derived from Rhizoctonia solani for H. muticus
hairy roots (Singh et al., 1994), and those derived from
Botrytis spec., Pythium aphanidermatum,
Rhodotorula rubra, Alternaria zinnae,
Colletotrichum gloeosporoides, Helminthosporium gramineum,
Sclerotinia sclerotiorum and Verticillium dahliae
for Papaver somniferum hairy roots (Eilert et al., 1985).
Meanwhile, examples of abiotic elecitors that have been applied
to hairy root cultures to elicit the production of secondary
metabolites include osmotic shock on H. muticus hairy
roots (Halperin and Flores 1997), and salicylate and methyl
jasmonate on Tanacetum parthenium hairy roots
(Stajokowska et al., 2002).
The mode of action of abiotic elicitors on plants has been
postulated in various ways, including through the release of an
endogenous elicitor compound from the plant cell (Moesta and
Grisebach 1981), through inhibition of the degradative turnover
of secondary metabolites (Yoshikawa 1978), and through the
involvement of a second messenger molecule that transmits
signals from the plasma membrane to trigger the transcription
and translation of enzymes (DiCosmo and Misawa 1985).
Electricity as an Elicitor
Elicitation has long been proposed as a potential strategy for
increasing the production of secondary metabolites by plant cell
or root cultures in vitro while they are being grown in
bioreactors (Dornenburg and Knorr 1995). There are significant
challenges, however, in employing elicitors, including: (1) the
labor required to prepare biotic elicitors from their biological
sources; (2) loss of capacity of biotic elicitors over time to
elicit the biosynthesis of desired secondary metabolites; (3)
potential adverse or toxic effects of biotic or abiotic
elicitors on the cell or root culture; and (4) difficulty of
separating or removing biotic or abiotic elicitors from the
culture once they have been added. While with respect to the
last point, UV light (an abiotic elicitor) provides the
convenience of lending itself to simply being turned on or off
as needed, the poor penetration of light through thick clumps of
hairy roots in a large-scale bioreactor constitutes a
significant challenge to subjecting all the roots in the
bioreactor uniformly to the elicitation process, especially when
the UV light is applied externally to the bioreactor. Inserting
UV lamps into the bioreactor interior, however, would waste
bioreactor space or volume, would add complexity to bioreactor
maintenance, cleaning, and sterile operation, and could
significantly interfere with the bioreactor’s hydrodynamic or
aerodynamic characteristics.
The use of electricity, however, as an abiotic elicitor obviates
the foregoing challenges. Similar to UV light, electricity
provides the convenience of lending itself to simply being
turned on or off as needed. Further, electricity would be
conducted much more efficiently than light through the thick
clumps of hairy roots in a large-scale bioreactor when the hairy
roots are immersed in their normal nutrient solution containing
electricity-conducting ions, allowing for all the roots in the
bioreactor to be subjected to the elicitation process in a
convenient manner. Also, implementing electrical elicitation
would only require two thin metal electrodes to be inserted
through the roots inside the bioreactor along the height of the
root bed. Alternatively, the electrodes or even metal plates
could be positioned against opposite inside walls of the
bioreactor if interference with the flow characteristics of the
bioreactor would be a concern.
The application of electricity in plant systems has been
traditionally focused on the effects of the presence of electric
fields or pulses on the following: (1) enhancement of the
proliferation rate of plant biomass (Rathore and Goldsworthy
1985); (2) increase in the rate and efficiency of plant
regeneration (Rathore and Goldsworthy 1985); (3) improvement of
the rate and success of seed germi-nation (Kazanova 1972); (4)
stimulation of protoplast division Rech et al., 1987); and (5)
enhancement of DNA synthesis in cultured plant protoplasts (Rech
et al., 1988). Takeda et al., (1988) correlated the membrane
potential of cultured carrot cells with their synthesis of
anthocyanin. They varied the membrane potential of the carrot
cells, however, by manipulating the concentration of K+
ions and by adding various levels of 2,4-D into the culture
medium, and not by subjecting the cells to an electric field.
Working with alternating current (AC) and direct current (DC)
and how they affect the production of secondary metabolites of
H. muticus hairy roots, Cuello and Yue (1998) and Johnson
and Cuello (1998) appear to be the first to investigate the
electrical elicitation of secondary metabolites from hairy root
cultures. Both studies employed a 6.3 cm x 6.3 cm x 5.1 cm
plexi-glass electrical elicitation box or chamber equipped with
two stainless-steel metal plates, serving as electrodes,
positioned against opposite inside walls of the box (Figure 6).
The hairy roots were placed inside the box and were immersed in
their normal nutrient solution before carrying out the
electro-elicitation process.
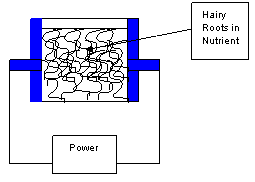
Figure 6.
Schematic of an electrical elicitation box equipped with
stainless-steel metal plates, serving as electrodes, placed
against opposite inside walls of the box.
A decidedly more comprehensive study on electrical elicitation
was recently conducted by Kaimoyo et al., (2008), using hairy
roots of pea (Pisum sativum, L, var. Little
Marvel) as the primary model system. Kaimoyo et al., (2008) used
the same elicitation box that Cuello and Yue (1998) and Johnson
and Cuello (1998) had used earlier. Trying different levels of
root biomass and electric current, their results showed that 2 g
of hairy roots exposed to 100 mA current for 1 h or 10 g of
hairy roots exposed to 30 or 50 mA current for 1 h produced very
similar levels of the secondary metabolite pisatin, which
averaged 32.1
±
3.9
mg g-1 FW.
This amount of pisatin was comparable to that obtained using
0.08 mM aqueous CuCl2 as an abiotic elicitor of de
novo pisatin biosynthesis (37
±
3.3 g g-1 FW). They also found that, though the
average length of the electro-elicited hairy roots six days
after treatment was slightly less than that for the control, the
rate of growth from six days on was the same as that of the
non-elicited hairy roots. And when these hairy roots were
re-elicited with 0.08 mM CuCl2 or a 30 mA current,
they produced similar amounts of secondary metabolites as
produced by hairy roots that had not been elicited previously.
Their gross morphology also did not differ from that of
non-elicited controls.
More interestingly, Kaimoyo et al., (2008) tested electrical
elicitation on other plant species known to synthesize
phytoalexins and other secondary metabolites upon elicitation by
various biotic and abiotic elicitors. Cell suspension cultures
of Arabidopsis (Arabidopsis thaliana L.) and
barrel medic (Medicago truncatula L.), roots of
chickpea (Cicer arietinum L.) and fenugreek (Trigonella
foenum-graecum L.), intact seedlings of sorghum (Sorghum
bicolor L.), Japanese pagoda tree (Sophora japonica
L.) and red clover (Trifolium pratense L.) were
electro-elicited at 30 mA current for 1 h. Secondary metabolites
were extracted 24 h later and compared by HPLC or HPLC coupled
to mass–spectrometry (HPLC-MS) with those extracted from
non-elicited tissues. Except for Arabidopsis and
sorghum, there were substantial increases in most of the
compounds whose biosynthesis had been shown previously to be
stimulated by elicitors. The resulting ratios of compound
produced with elicitation to compound produced by the
non-elicited control were: 2.8 for medicarpin as produced by
fenugreek roots; 4.3 for formononetin as produced by chickpea
roots; 44.0 for maackiain, 5.0 for medicarpin and 15.6 for
formononetin as produced by red clover seedlings; 34.5 for
afrormorsin, 168.1 for medicarpin, 7.0 for irisolidone, 7.6 for
formononetin, 3.6 for naringenin, 3.8 for hispidol, 3.5 for
tricin and 5.2 for isoflav-3-ene as produced by barrel medic
cells in suspension.
The authors noted that, in addition to the expected compounds,
the HPLC profiles of the extracts from many of the plants showed
the presence of other previously undetected secondary
metabolites. For instance, extracts of chickpea roots showed a
large increase in formononetin, maackiain and medicarpin as well
as over 20 other metabolites. The production of secondary
metabolites by cell suspension cultures of barrel medic showed
an even greater increase in the number of compounds as 55
compounds showed at least a 2-fold increase in the
electro-elicited tissue compared to the non-electro-elicited
controls. In the cell suspension cultures of Arabidopsis the
expected accumulation of camalexin was not observed. Silver
nitrate, previously reported to induce camalexin biosynthesis in
leaf tissue, was used as a positive control, but no camalexin
was obtained from silver nitrate-treated Arabidopsis cell
suspensions. However, two peaks with residence time or RT = 9
min and 13 min, respectively were observed in the HPLC
chromatogram of extracts from electro-elicited Arabidopsis
cell suspension cultures that were absent in non-elicited
cell suspensions. In sorghum seedlings, several different
metabolites were detected in the extracts of both elicited and
non-elicited tissue, but the differences in the amounts of these
compounds were not significant.
The study by Kaimoyo et al., (2008) clearly indicated that
electric current constitutes a general abiotic elicitor of plant
secondary metabolites. As with other abiotic elicitors, the
mechanism of action of electricity as elicitor on plants could
potentially involve the release of an endogenous elicitor
compound from the plant cell (Moesta and Grisebach 1981), the
inhibition of the degradative turnover of secondary metabolites
(Yoshikawa 1978), and/or the involvement of a second messenger
molecule that transmits signals from the plasma membrane to
trigger the transcription and translation of enzymes (DiCosmo
and Misawa 1985).
Electrical Elicitation of
Hyoscyamus muticus Hairy Roots
In our own study, 14-day-old H. muticus hairy roots were
subjected for 1 h to 12 mA, 67 mA and 158 mA of direct current
(DC) and to 11 mA, 28 mA, and 288 mA of 60-Hz alternating
current (AC). Weighing an average of 16.3 g per treatment, the
roots were treated for alkaloid extraction by the method of
Kamada et al., (1986), and alkaloid extracts dissolved in
methanol were assayed using HPLC (Shimadzu) equipped with
SIL-10A autoinjector and SPD-M10A photodiode array detector.
Samples were injected at a volume of 20 mL and were separated
isocratically in a Nova-Pak C18 (Waters) steel column (3.9 x 150
mm) using a mobile phase of 12.5% (v v-1)
acetonitrile and 87.5% (v/v) aqueous phosphoric acid (0.3% v/v),
adjusted to pH 2.2 with triethylamine, at a flow rate of 0.8 mL
min-1.
While the average pH of the roots’ B5 liquid medium did not
significantly change after elicitation, the medium’s average
electrical conductivity increased significantly for all
treatments after elicitation. For the DC treatments, the average
electrical conductivity rose from 1.8 to 10.0 mS cm-1
for 12 mA, 0.9 to 8.8 mS cm-1 for 67 mA, and 0.8 to
19.3 for 158 mA. For the AC treatments, the average electrical
conductivity rose from 1.5 to 7.8 mS cm-1 for 11 mA,
1.6 to 6.6 mS cm-1 for 28 mA, and 0.9 to 27.8 for 288
mA.
The resulting HPLC chromatograms at 215 nm of the roots’
alkaloid extracts showed pronounced differences between those
for the elicited treatments and those for the unelicited control
(Figure 7). Compound I (RT = 8.6 min) was significantly elicited
or overproduced when the roots were electro-elicited using
either AC or DC elicitation (Table 2). Indeed, the ratios of the
level of Compound I in an elicited treatment to that in the
control ranged from 11.0 to 13.5 for the AC treatments, while
those for the DC treatments ranged from 3.8 to 14.4. By
contrast, however, the levels of Compound II (RT = 9.8 min)
remained statistically indistinguishable between the elicited AC
or DC treatments and the unelicited control (Table 2). And,
interestingly, the synthesis of Compound III (RT = 16.0) was
significantly inhibited by both AC and DC electrical
elicitation.
Thus, the results of this study showed that in the case of H.
muticus hairy roots, electrical elicitation significantly
overproduced compound I, significantly inhibited compound III,
and had no appreciable effect on the level of compound II. It is
likely that the employment of electrical elicitation to a
specific plant species will have these three general effects on
the plant’s specific sets of secondary metabolites.
EBB-AND-FLOW BIOREACTOR
REGIME AND ELECTRICAL ELICITATION
Based on the foregoing sections, the ebb-and-flow regime and
electrical elicitation constitute two novel and promising
effective strategies for realizing the large-scale production of
secondary metabolites from hairy roots growing in scaled-up
bioreactors. Employing the ebb-and-flow regime would provide the
forced-convective action that is needed to overcome the
mass-transfer resistances offered by the stagnant external
boundary layers and/or dead zones which normally exist in the
high-density hairy-root bioreactor environment – both in
liquid-phase and in gas-phase bioreactors. Meanwhile, employing
electrical elicitation could significantly overproduce valuable
secondary metabolites produced by the hairy roots. Combining the
two strategies could certainly help achieve the economic
feasibility of the large-scale production from plant hairy roots
of specific secondary metabolites.
 
  
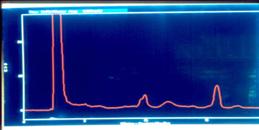

 
   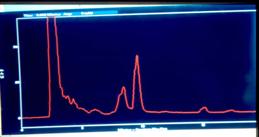
Figure 7.
Replicate HPLC chromatograms at 215 nm of the alkaloid extracts
from Hyoscyamus muticus hairy roots for the control
(upper) and for an electro-elicited treatment [12 mA DC] (low).
The x-axis is retention time (RT) and the y-axis is absorbance
units. Compound I has RT = 8.6 min, Compound II has RT = 9.8
min, and Compound III has RT = 16.0 min.
Table 2.
Average levels of compound I (RT = 8.6 min) and compound II (RT
= 9.8 min) based on the HPLC chromatograms at 215 nm of the
alkaloid extracts from Hyoscyamus muticus hairy roots.
The letters “a”, “b” and “c” denote statistical differences
between the control and the AC and DC electro-elicitation
treatments.
|
Compound I |
Compound II |
Average peak Area
(1000x) |
Elicited/Control |
Average peak Area
(1000x) |
Elicited/Control |
Unelicited control |
31.3a |
1.0 |
292.0a |
1.0 |
Elicited AC treatments |
|
|
|
|
11 mA |
423.4b |
13.5 |
298.4a |
≈ 1.0 |
28 mA |
366.8b |
11.7 |
345.1a |
≈ 1.0 |
288 mA |
346.5b |
11.0 |
325.5a |
≈ 1.0 |
Elicited DC treatments |
|
|
|
|
12 mA |
420.8b |
13.4 |
514.7a |
≈ 1.0 |
67 mA |
453.5b |
14.4 |
342.8a |
≈ 1.0 |
158 mA |
120.0c |
3.8 |
359.1a |
≈ 1.0 |
REFERENCES CITED
Buitelaar RM,
Leenan EJTM, Geurtsen G, de Groot AE, Tramper J (1993).
Effects of the addition of XAD-7 and of elicitor treatment on
growth, thiopene production, and excretion by hairy roots of
Tagetes patula. Enzyme Microb Technol 15: 670-676
Cuello JL,
Walker PN, Curtis WR
(2003a). Design of ebb-and-flow biorector (EFBR) for immobilized
hairy root cultures, Part I: Preliminary design models and culture
parameters. Trans ASAE 46: 1457-1468
Cuello JL,
Walker PN, Curtis WR
(2003b). Design of ebb-and-flow biorector (EFBR) for immobilized
hairy root cultures, Part II: Growth studies and model
verifications. Trans ASAE 46: 1469-1476
Cuello JL, Yue LC
(1998). Electrical elicitation of secondary metabolites from in
vitro root cultures. The University of Arizona Office of the
Vice President for Research Small Grant Program Report. The
University of Arizona, Tucson, AZ
Cuello JL
(1994). Design and scale up of ebb-and-flow bioreactor (EFBR) for
hairy root cultures. PhD dissertation, University Park, Penn State
University, State College, PA
Cuello JL, Walker PN,
Curtis WR
(1991). Ebb-and-flow bioreactor for hairy root cultures. ASAE Paper
No. 91-7528. St. Joseph, Mich: ASAE
Darville AG, Albershein P
(1984). Phytoalexins and their elicitors – a defense against
microbial infection in plants. Ann Rev Plant Physiol 35:
243-275
Dawson RF
(1942). Nicotine synthesis in excised tobacco roots. Amer J Bot
29: 813-815
DiIorio AA, Cheetham RD, Weathers PJ (1992). Growth of transformed roots
in a nutrient mist biorector: Reactor performance and evaluation.
App Microbiol Biotechnol 37: 457-462
Dornenburg H, Knorr D
(1995). Strategies for the improvement of secondary metabolite
production in plant cell cultures. Enzyme Microb Technol 17:
674-684
Eilert U, Kurz WGW, Constabel F
(1985). Stimulation of sanguinarine accumulation in Papaver
somniferum cell cultures by fungal elicitors. J Plant Physiol
119: 65-76
Flores HE, Dai YR, Cuello JL,
Maldonado-Mendoza IE, Loyola-Vargas VM
(1993). Green roots: Photo-synthesis and photoautotrophy in an
underground plant organ. Plant Physiol 101: 363-37
Flores HE, Curtis WR
(1991). Approaches to understanding and manipulating the
biosynthetic potential of plant roots. Proc NY Acad Sci 655:
188-209
Flores HE
(1987). Use of plant cells and organ culture in the production of
biological chemicals. In Biotechnology in Agricultural Chemistry,
Washington, DC: ACS Symposium Series
Halperin SJ, Flores HE
(1997). Hyoscyamine and praline accumulation in water-stressed
Hyoscyamus muticus hairy root cultures. In Vitro Cell Dev Biol
Plant 33: 240-244
Hamill JD, Parr AJ, Rhodes M, Robins RJ, Walton NJ (1987). New routes to
plant secondary products. Bio Technology 5: 800-804
Hilton MG, Rhodes
MJC
(1990). Growth and hyoscyamine production of hairy root cultures
of Datura stramonium in a modified stirred tank reactor.
Applied Microbiol Biotechnol 33: 132-138
Johnson M, Cuello JL
(1998). Overproduction of plant-produced chemicals from in vitro
root cultures. 1997-1998 The Spirit of Inquiry. Abstract,
Undergraduate Research, The University of Arizona Honors Center.
Tucson, AZ
Kaimoyo E, Farag MA,
Sumner LW, Wasmann C, Cuello JL, Van Etten H (2008).
Sub-lethal levels of electric current elicit the biosynthesis of
plant secondary metabolites. Biotechnol Prog 24: 377 -384
Kamada H, Okamura N,
Satake M, Harada H, Shimomura K
(1986). Alkaloid production by hairy root cultures in Atropa
belladonna. Plant Cell Reports 5: 239-242
Kanokwaree K, Doran PM
(1998). Application of membrane tubing aeration and
perfluorocarbon to improve oxygen delivery to hairy root cultures.
Biotechnol Prog 14: 479-486
Kanokwaree K, Doran PM
(1997). The extent to which external oxygen transfer limits growth
in shake flask culture of hairy roots. Biotechnol Bioeng 55:
520-526
Kazanova ZM
(1972). After-sowing processing of spring wheat seeds in electrical
constant current. Electr Process Mat. 4: 71-72
Kim YJ, Weathers PJ,
Wyslouzil B
(2002). Growth of Artemisia annua hairy roots in liquid and
gas-phase reactors. Biotechnol Bioeng 80: 454-464
Kondo O, Honda H,
Taya M, Kobayashi T
(1989). Comparison of growth properties of carrot hairy root in
various bioreactors. Applied Microbiol Biotechnol 32: 291-294
Kwok KH, Doran PM
(1995). Kinetic and stoichiometric analysis of hairy roots in
segmented bubble column reactor. Biotechnol Prog 11: 429-435
Medina-Bolivar F, Condori
J, Rimando AM, Hubstenberger J, Shelton K, O’Keefe SF, Bennett S,
Dolan MC
(2007). Production and secretion of resveratrol in hairy root
cultures of peanut. Phytochem 68: 1992-2003
Medina-Bolívar F, Cramer C
(2004). Production of recombinant proteins by hairy roots cultured
in plastic sleeve bioreactors. In Methods in Molecular Biology:
Recombinant Gene Expression. 2nd ed.
Paulina Balbás and Argelia Lorence
(eds). Molecular Biology Series. Humana Press, Totowa, 535 pp
Medina-Bolivar F, Wright
R, Funk V, Sentz D, Barroso L, Wilkins TD, Petri W, Cramer CL (2003). A non-toxic
lectin for antigen delivery of plant-based mucosal vaccines. Vaccine
21: 997-1005
Ramakrishnan D, Curtis WR
(2004). Trickle-bed root culture bioreactor design and scale up:
growth, fluid dynamics and oxygen mass transfer. Biotechnol Bioeng
88: 248-260
Ramakrishnan D, Curtis WR
(1995). Elevated meristematic respiration in plant root cultures:
implications to reactor design. J Chem Eng Jpn 28: 491-493
Rathore KS, Goldsworthy A
(1985). The electrical control of growth in plant tissue cultures:
the polar transport of auxin. J Exp Bot 36: 1134-1141
Rech EL, Ochatt SJ, Chand
PK, Davey MR, Mulligan BJ, Power JB
(1988). Electroporation increases DNA synthesis in cultured plant
protoplasts. BioTechnology 6: 1091-1093
Rech EL, Ochatt SJ, Chand
PK, Power JB, Davey MR
(1987). Electroenhancment of division of plant-protoplast-derived
cells. Protoplasma 141: 169-176
Rhodes MCJ, Hilton M, Parr
AJ, Hamill JD, Robins RJ
(1986). Nicotine production by hairy root cultures of Nicotiana
rustica: Fermentation and product recovery. Biotechnol Letters
8: 415-420
Shiao T, Doran PM
(2000). Root hairiness: Effect on fluid flow and oxygen transfer in
hairy root cultures. J Biotechnol 83: 199-210
Shiao T, Ellis MH, Dolferus R, Dennis ES, Doran PM
(2002). Overexpression of alcohol dehydrogenase or pyruvate
decarboxylase improves growth of hairy roots at reduced oxygen
concentrations. Biotechnol Bioeng 77: 455-461
Singh G, Reddy GR, Curtis
WR
(1994). Use of binding measurements to predict elicitor dosage
requirements for secondary metabolite production from root cultures.
Biotechnol Prog 10: 365-371
Stojakowska AJM, Kisiel W
(2002). Salicylate and methyl jasmonate differentially influence
diacetylene accumulation pattern in transformed roots of feverfew.
Plant Sci 163: 1147-1152
Takeda J, Senda M, Ozeki
Y, Komamine A
(1988). Membrane potential of cultured carrot cells in relation to
the synthesis of anthocyanin and embryogenesis. Plant Cell Physiol
29: 817-824
Taya M, Yoyama A, Kondo O,
Kobayashi T
(1989). Growth characteristics of plant hairy roots and their
cultures in bioreactors. J Chem Engin Jpn 22: 84-89
Weathers PJ,
Wyslouzii BE, Wobbe KK, Kim YJ, Yigit E
(1999). Workshop on Bioreactor Technology: The biological response
of hairy roots to O2 levels in bioreactors. In Vitro Cell
Develop Biol Plant 35: 286-289
Williams RC, Doran
PM
(2000). Hairy root culture in a liquid-dispersed bioreactor:
Characterization of spatial heterogeneity Biotechnol Prog 16:
391-401
Yu S, Doran PM
(1994). Oxygen requirements and mass transfer in hairy-root
culture. Biotechnol Bioeng 44: 880-887
Accepted for
publication: 18 October 2008
|