Expression of a ricin B:F1:V fusion protein
in tobacco hairy roots: steps toward a novel
pneumonic plague vaccine
Bonnie J. Woffenden1,#, Luis H. Ñopo2,
Carole L. Cramer2,3, Maureen C. Dolan2,
and Fabricio Medina-Bolivar2,3,*
1
Department of Plant Pathology, Physiology and
Weed Science, Virginia Tech, Blacksburg, VA.
#
Current address: Science and Technology
Corporation at Edgewood Chemical Biological Center,
US ARMY RDECOM, Aberdeen Proving Ground, Edgewood, MD.
2
Arkansas Biosciences Institute, Arkansas State University,
Jonesboro, AR.
3
Department of Biological Sciences, Arkansas State
University, Jonesboro, AR.
*Corresponding author: F. Medina-Bolivar; email:
fmedinabolivar@astate.edu
Keywords:
plague vaccine, hairy roots, ricin B, tobacco
ABSTRACT
Recombinant
subunit vaccines consisting of the F1 and V antigens of
Yersinia pestis have shown remarkable promise for protection
of animals and humans against bubonic and pneumonic plague.
Previously we demonstrated that intranasal administration of a
recombinant protein consisting of the ricin B chain (RTB) fused
with an antigen purified from transgenic Nicotiana tabacum
(tobacco) hairy root cultures was highly effective in
stimulating a mucosal antibody response in mice (Medina-Bolivar
et al., 2003). In the current study, transgenic tobacco plants
and hairy roots were engineered to express a recombinant protein
consisting of a hexahistidine-tagged RTB fused to tobacco
sequence-optimized F1 and V genes (His:RTB:F1:V). Northern blot
analysis of crude leaf extracts revealed that twenty-eight
transgenic plant lines expressed a transcript of the predicted
mRNA size detectable with the RTB probe. An RTB functional ELISA
based on asialofetuin-binding activity was used to screen crude
leaf protein extracts from these transgenic plants and select
the two lines with the highest levels of fuctional RTB lectin
activity. Analysis of the tissue localization of the recombinant
mRNA in transformed plants and hairy roots developed from these
plants suggested that His:RTB:F1:V expression levels were higher
in hairy roots than in any tissue of the original transgenic
plants. Asialofetuin-binding ELISA of transgenic hairy root
extracts confirmed expression of functional RTB-containing
fusion protein. Immunoblotting of lactose affinity-purified
protein from hairy root lines using anti-RTB, anti-His-tag, and
anti-V antibodies detected a protein of approximately 91 kDa,
the predicted size of glycosylated His:RTB:F1:V. Purified
His:RTB:F1:V protein will be used for intranasal immunization in
mice, in which stimulation of mucosal immunity will be a first
step towards an improved pneumonic plague vaccine.
INTRODUCTION
The causative agent
of bubonic and pneumonic plague is the bacterium Yersinia
pestis. While this scourge is infamous for its capacity to
cause pandemic and epidemic plague disease, naturally occurring
smaller eruptions of both plague forms continue to occur in
scattered geographic pockets across the globe (Bertherat et al.,
2005; Mudur 1995). Y. pestis ranks among the top five
potential bioweapon threats due to the rapid contagion of
pneumonic plague through spread of aerosolized bacteria from
infected patients and near 100% lethality of untreated pneumonic
plague cases within 24 hours of infection. Although antibiotics
have proven effective in treating most known strains of Y.
pestis, multidrug resistant plague strains have recently
emerged (Galimand et al., 1997; Guiyoule et al., 2001).
Moreover, no U.S.-licensed plague vaccine exists. In 1999,
usage of the USP whole cell, killed plague vaccine that had been
used worldwide for over a hundred years was discontinued in the
U.S. due to concerns over significant side effects and
questionable vaccine efficacy (Cohen et al., 1967; Meyer 1970;
Williamson et al., 1997). These issues underscore the
importance of sustained investment in the development of a
highly effective vaccine for plague now.
Recombinant subunit
vaccines provide a promising avenue toward protection against
both bubonic and pneumonic plague and are expected to lack the
potential for the undesirable side effects contributed by either
killed or attenuated whole organism vaccines. Two Y. pestis
virulence factor proteins, F1 (Fraction I, encoded by caf1)
and V (Virulence, encoded by lcrV), have dominated recent
plague vaccine research and development efforts. F1 is a
structural protein of the plague capsular antigen expressed on
the bacterial cell surface (Titball et al., 1997) and prevents
phagocytosis by host cells (Du et al., 2002). V is a secreted
protein that participates with the Type III secretion pathway in
introduction of a number of Y. pestis outer proteins into
host cells (Fields et al., 1999). Subunit vaccines comprised of
both the F1 and V proteins have been demonstrated in animal
models to confer protection against both the bubonic and
pneumonic forms of disease and also have been well-tolerated in
humans (Alpar et al., 2001; Anderson et al., 1998; Glynn et al.,
2005; Heath et al., 1998; Jones et al., 2000, 2003, 2006;
Williamson et al., 1997, 2001, 2005, 2007). Recent reports
demonstrate the potential of subunit F1 and V plague vaccines
produced in plant model systems to stimulate relevant antibody
production in mice (Alvarez et al., 2006) and to protect against
lethal plague challenge in a primate model (Mett et al., 2007).
The premise of this
study is that a tobacco-produced F1:V subunit vaccine that
includes a fusion partner to target the purified protein to the
mucosa of vaccinated individuals may better enhance protection
against pneumonic plague compared to non-mucosal formulations,
while avoiding the harmful side effects commonly associated with
many chemical adjuvants. Previously, we demonstrated that the
non-toxic lectin subunit of ricin, ricin B chain (RTB), is
useful as a mucosal carrier/adjuvant (Medina-Bolivar et al.,
2003). Intranasal administration of recombinant RTB:antigen
fusion protein purified from transgenic tobacco hairy root
cultures was highly effective in stimulating an antigen-specific
mucosal antibody response in mice (Medina-Bolivar et al.,
2003). The current report demonstrates successful tobacco-based
expression and purification of a novel genetic fusion of the
Y. pestis F1 and V genes with RTB (His:RTB:F1:V), a novel
mucosal adjuvant:antigen strategy.
RESULTS
Development of the
gene construct for tobacco-based expression of a candidate
subunit vaccine against pneumonic plague
The transgene construct designed for expression in tobacco of
the candidate subunit plague vaccine consisting of RTB in fusion
with the Y. pestis F1 and V proteins is shown in Figure
1. To direct constitutive expression of the transgene, we
utilized the chimeric, synthetic, super-promoter:TEV (Ni et al.,
1995; Lee et al., 2007), which consists of activator and
promoter sequences from the octopine synthase and mannopine
synthase genes of Agrobacterium tumefaciens in fusion
with the tobacco etch virus translational enhancer. The
super-promoter has yielded high-level expression of transgenes
in tobacco (Ni et al., 1995; Lee et al., 2007). Because we
previously observed that secretion of the expressed transgene
product can provide advantages for protein recovery and
purification (Medina-Bolivar et al., 2003; Reed et al., 2005),
the signal peptide (Pat) from the potato patatin gene was used
to direct ER targeting and secretion of the recombinant protein.
In addition,
targeting into the secretory pathway is needed for glycosylation
of RTB. A hexahistidine tag
(His) was included to provide a facile method for detection and
protein purification from transgenic plants. Due to the
demonstrated ability of the non-toxic subunit of RTB to
stimulate mucosal immune responses to a genetically fused model
antigen (Medina-Bolivar et al., 2003), RTB was included to act
as a mucosal adjuvant for the F1:V plague antigens. To
circumvent potential problems with expression of the prokaryotic
F1 and V in a plant system, several modifications of the
bacterial genes were designed, and the coding region of each
gene was commercially synthesized and used in the assembly of
the corresponding transgene constructs. The F1 and V gene
sequences were optimized for consideration of tobacco codon
usage preferences, elimination of cryptic eukaryotic RNA
processing signals (including potential premature
polyadenylation sites, intron splice sites, and RNA instability
elements), and removal of potential N-glycosylation sites. The
synthetic F1 and V genes were expected to contribute 15 kDa and
37 kDa to the fusion protein product. The gene construct
super-promoter:TEV:Pat:His:RTB:F1:V referred as pP10 was used to
generate transgenic tobacco plants and hairy roots for this
study.
Generation and
screening of transgenic tobacco plants and hairy roots
The binary vector pP10 containing the transgene was mobilized
into Agrobacterium tumefaciens, which was then used to
transform Nicotiana tabacum cv. Xanthi according to a
petiole-inoculation method previously described (Medina-Bolivar
et al., 2003; Medina-Bolivar and Cramer, 2004). The transformed
tobacco plants that were regenerated (28 lines) were
phenotypically normal. Hairy root lines were developed
from all plant lines containing the transgene construct by
methods described before (Medina-Bolivar and Cramer, 2004).
As an initial genetic screen of the regenerated transformants,
PCR analysis of genomic DNA extracted from the putative
transgenic plants was conducted using RTB-specific primers.
Figure 2 shows that the predicted 800 bp PCR fragment
corresponding to RTB cDNA was observed in
all
pP10-transformed plant lines and was not generated from
non-transformed tobacco cv. Xanthi genomic DNA.

Figure 1. DNA construct utilized for expression of
Pat:His:RTB:F1:V in transgenic tobacco plants and hairy roots.
Shown are the chimeric super-promoter:TEV (pE1802 backbone, Ni
et al., 1995), patatin signal peptide (Pat) used to target the
fusion protein for secretion/glycosylation, the hexahistidine
tag (His; HHHHHH) included as a purification and detection tag,
ricin B chain (RTB), and the synthetic F1 (sF1) and V (sV)
genes, as well as the restriction sites used for cloning
purposes.
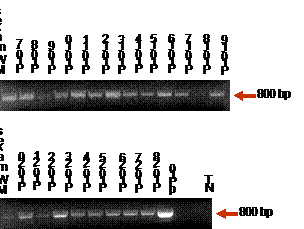
Figure 2.
PCR screening of selected putative transgenic plants. RTB-specific
primers were used in PCR of genomic DNA isolated from leaves of
22 putative P10 transformants, non-transformed tobacco (NT), and
from a positive control plasmid (pP10). The migration position
of the expected 800 bp PCR fragment is indicated by the arrows.
To rapidly identify
transgenic tobacco plants expressing high levels of RTB:F1:V
fusion protein we utilized an asialofetuin-binding ELISA. The
lectin function of RTB enables binding to the galactose-terminated
glycoprotein asialofetuin with approximately 1000-fold greater
affinity compared to RTB’s binding affinity for galactose.
Previously, we described this ELISA to screen transgenic tobacco
plants expressing RTB (Reed et al., 2005). Following this
method, all P10 plants were screened for recombinant protein
expression. ELISA testing of crude leaf extracts from P10 plant
lines revealed in three experiments that lines P10-13 and P10-19
express the highest levels of recombinant protein competent to
bind asialofetuin. Figure 3 shows the results of one experiment
for 7 representative plant lines, including P10-13 and P10-19,
which yielded about 0.025 and 0.015 ng of RTB equivalents per µg
total leaf protein, respectively. These values represented 45
and 44 ng RTB equivalents per g FW leaf, respectively. The lines
P10-13 and P10-19, including plants and hairy roots, were
selected for further studies.
Following
identification of the transgene by PCR, integration of the P10
transgene into the genomic DNA of lines P10-13 and P10-19 was
verified by Southern blotting (Figure 4). Genomic DNA was
digested with SacI, as this restriction enzyme has only
one recognition site within the T-DNA and will help to identify
the copy number of inserts in the transgenic plants using an RTB
probe. All bands detectable with this probe are likely larger
than 8 kb, based on the migration of DNA size markers. Plant
lines P10-13 and P10-19 show distinct banding patterns,
indicating that they represent two independent transgenic lines.
Line P10-13 exhibits two insertion loci, while line P10-19 shows
transgene insertion at 4-5 loci. Hairy root lines developed
from these cultures also show the same insertion pattern as
their parental plant lines (not shown). The RTB-containing
positive control plant (line R6-2 developed by Medina-Bolivar et
al., 2003) shows the expected pattern of transgene loci (Maureen
Dolan, unpublished results).
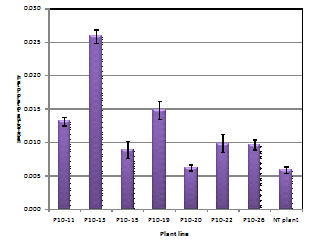
Figure 3.
Functional ELISA of P10 protein expression in transgenic plants.
An asialofetuin-binding ELISA of crude extracts was conducted
with 100 µg of total protein to quantify P10 protein expression.
Crude leaf extracts from P10 plant lines (11, 13, 15, 19, 20,
22, and 26) and a non-transformed tobacco sample (NT plant) were
assayed for asialofetuin-binding activity. Error bars shows the
variation between three repetitions. Statistical analysis showed
significant difference in yield of functional the P10 protein in
every transgenic plant compared to the control, except for
P10-20 (Dunnett’s t Test,
α
= 0.05).
Several hairy root
lines were developed from plants P10-13 and P10-19. However,
hairy roots from plant P10-19 (R1, R2, R4 and R5) showed better
growth in liquid culture and these were further analyzed.
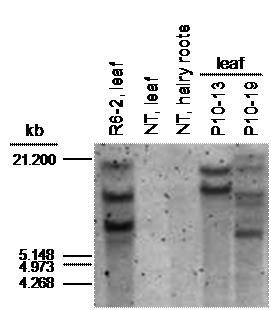
Figure 4. Southern blotting of selected transgenic plant lines.
Genomic DNAs isolated from plant lines P10-13 and P10-19,
non-transformed tobacco leaf and hairy roots (NT), and a
previously characterized positive control line expressing a
different RTB-containing transgene (R6-2) were digested with
SacI, and processed for Southern blotting with an RTB-specific
probe.
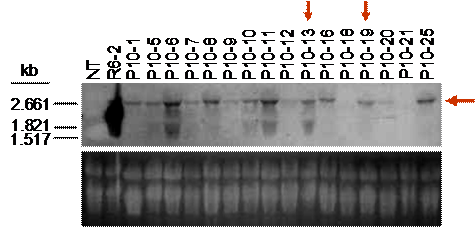
Figure 5.
Northern blot of transgenic plant leaf P10 mRNA levels.
Total RNA (20
μg)
from leaves of P10 plant lines, non-transformed tobacco (NT),
and a previously characterized RTB-containing transgenic line
(R6-2) was processed for Northern blotting with an RTB-specific
probe (top panel). The arrow to the right of the blot indicates
the migration position of the 2.9 kb P10 transcript. Arrows
above the blot denote two plant lines (P10-13, P10-19) studied
in greatest detail herein. Ethidium-bromide staining of the gel
prior to RNA transfer demonstrated equal RNA loading per lane
(bottom panel).
Basal expression
of P10 mRNA in plants and hairy roots
The basal level of
P10 mRNA expression in leaves of the transgenic plants was
examined by Northern blotting using a probe specific for RTB.
The P10 mRNA is predicted to be at least 2.5 kb, and an
approximately 2.9 kb transcript was detected with the RTB probe
in a total of 22 P10 plant lines. Figure 5 shows a
representative RNA blot of 16 P10 lines, two of which did not
express detectable levels of P10 mRNA. Relative to the mRNA
level observed for the transgene in the positive control R6-2
line, which expresses a different RTB-containing construct,
fourteen of the P10 lines shown express very low levels of P10
mRNA. Somewhat surprisingly, the abundance of P10 mRNA in most
of the different lines was relatively equivalent, including
lines P10-13 and P10-19 which showed the highest levels of
asialofetuin-binding ELISA. These results were confirmed by
Northern blotting using an F1:V probe (data not shown).
Tissue localization
of transgene mRNA by Northern blotting of young leaves, mature
leaves, stem, and roots from plant line P10-13 revealed that the
P10 transcript is expressed ubiquitously but is not highly
abundant in any organ. The highest levels were observed in
roots, whereas the transcript was expressed at very low levels
in stem, and was barely detectable in either young or mature
leaves (data not shown). This experiment confirms that leaf P10
mRNA levels are generally low, as noted during the initial leaf
RNA blot (Figure 5). This observation that leaf P10 mRNA levels
are low and basal root levels are moderately higher suggested
that the hairy root culture expression system may be better
suited than whole plants for production of P10 using the
super-promoter.
To assess the levels
of P10 transcript in hairy root lines derived from plant line
P10-19 total RNA from lines P10-19 R1, R3, R4, and R5 was
analyzed by Northern blotting. The P10 transcript was expressed
at very similar levels in each of the P10-19 hairy root lines
tested (Figure 6), while those levels were significantly higher
than those observed in the leaf samples (data not shown).
Asialofetuin-binding
ELISA of hairy root protein extracts
In our previous
asialofetuin-binding ELISA of leaf protein extracts from
transgenic plants, two lines P10-13 and P10-19 were selected as
the highest expressors of functional P10 protein. In addition,
the tissue localization of P10 mRNA showed that roots had higher
basal levels of RNA than leaves, suggesting that hairy roots
could also yield higher levels of the recombinant protein P10
protein. To assess for functional P10 protein, hairy roots
lines P10-19 R1, R3, R4 and R5, were analyzed using this ELISA.
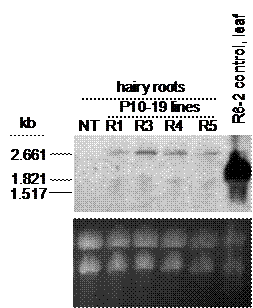
Figure 6.
Transgene mRNA expression in hairy root lines derived from
P10-19 plant.
Total RNA extracted from hairy root lines generated from plant
line P10-19 (R1, R3, R4, R5), from leaf and hairy roots of
non-transformed tobacco (NT), and from leaf of a positive
control line (R6-2) was processed for Northern blotting using an
RTB-specific probe (top panel). The bottom panel shows the
ethidium-bromide stained gel prior to RNA transfer, indicating
equal RNA loading per lane.
Figure 7 shows a
representative of two independent experiments in which the
levels of functional recombinant protein observed in hairy root
tissue (lines P10-19 R1, R3, R4 and R5) were at least 10-fold
greater than the levels observed in leaf tissue of the P10-19
plant line (Figure 3). The R1 and R5 hairy root lines produced
299 and 244 ng RTB equivalents/g FW root, respectively. No
functional P10 protein was detected in the medium of any of the
root lines therefore only extracts from hairy root tissue were
further analyzed by Western blotting.
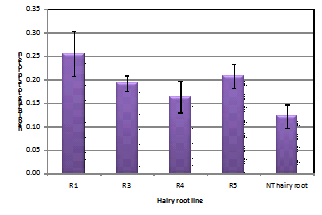
Figure 7.
Functional ELISA of P10 protein expression in transgenic hairy
root lines.
An asialofetuin-binding ELISA of crude extracts was conducted
with 100 µg of total protein to quantify P10 protein expression
in hairy roots derived from plant P10-19 (R1, R3, R4, and R5); a
non-transformed tobacco hairy root sample was also considered
(NT hairy root). Error bars shows the variation between three
repetitions. Statistical analysis showed significant difference
in the yield functional P10 protein for lines R1 and R5 compared
to the control; the other lines didn’t shown this difference (Dunnett’s
t Test,
α
= 0.05).
Purification of P10 protein from hairy roots and detection by
Western blotting
Crude extracts of P10-19 hairy root lines R1, R4, and R5 were
subjected to lactose affinity chromatography to purify P10
protein based on the carbohydrate-binding ability of RTB.
Western blotting of the affinity-purified samples revealed the
presence of an approximately 91 kDa protein detectable with
antibodies specific for RTB, the His-tag, and V antigen (Figures
8A, 8B and 8C, respectively) in all P10 hairy root lines
tested. This product is consistent with the predicted size of
fully intact recombinant P10 protein, glycosylated at the two
potential N-glycosylation sites within RTB. An approximately 90
kDa doublet was observed in P10 hairy root lines R1, R4, and R5
using the His-tag-specific antibody (Figure 8B). The bottom
band of the doublet is predicted to be P10 protein, whereas the
upper band co-purifying in much lower abundance is also detected
in the non-transgenic control. Immunoblotting with the anti-RTB
antibody detected four likely P10 degradation products (three in
the 35 kDa range, and one of nearly 50 kDa) in varying
quantities in the purified samples from the different P10 lines
but not in the non-transformed control (Figure 8A). Two to three
probable P10 degradation products were observed from P10 hairy
roots in the His-specific blot (Figure 8B), which also revealed
four additional non P10-derived bands that are also evident in
the non-transformed control. Detection of the 91 kDa P10
protein by the RTB and His-tag antibodies has been replicated
several times, while the profile of observed degradation
products has varied slightly between experiments. The
V-specific antibody detected a doublet of approximately 91 kDa
and one in the 35 kDa range in the purified sample from the
P10-19, R5 hairy root line. Co-purifying proteins in the
purified sample of the non-transformed line were detected.
However, a unique protein of 91 kDa was only observed in samples
from the transgenic P10-19, R5 hairy root (Figure 8C).
DISCUSSION
In this study, a DNA construct including the F1 and V antigens
of Yersinia pestis fused to the innovative, potential
mucosal adjuvant protein, RTB, was successfully expressed in
tobacco with the goal of developing a novel, recombinant
pneumonic plague vaccine (P10 protein = His:RTB:F1:V).
We predict that the ability of RTB to bind galactose present at
mucosal surfaces may be crucial to its function as a mucosal
adjuvant. Therefore, we initially used a functional ELISA that
tests the ability of binding to the galactose terminated
glycoprotein, asialofetuin, to screen for transgenic tobacco
lines with high levels of P10 protein. Initial results from
more than 20 P10 plant lines assayed using this ELISA identified
two lines P10-13 and P10-19 as the highest expressors of P10
protein in leaves. Interestingly, the levels of functional P10
protein in line P10-19 were at least 10-fold greater in hairy
roots than in leaves. These results are consistent with the RNA
blots which showed that transgenic plant roots (not shown) and
hairy roots express higher P10 transcript levels than leaf.
Though plant line P10-13 outperformed the others in three
independent ELISA experiments using leaf extracts, P10-13 hairy
root lines were discarded due to an undesirable growth
phenotype, and subsequent analyses focused on hairy roots
derived from the P10-19 plant. It is formally possible that
plant lines other than P10-13 and P10-19 expressed a higher
overall level of recombinant protein that was not competent to
bind asialofetuin under the conditions of this assay, and also
possible that overall expression levels may emerge as more
important than carbohydrate-binding ability. Nevertheless, we
selected several P10-19 hairy root lines for further analysis.
Four P10-19 hairy roots lines (R1, R3, R4, R5) yielded fairly
comparable RTB equivalents in the asialofetuin-binding ELISA,
and lines R1, R4, and R5 were subsequently analyzed for P10
protein expression by Western blotting.
Surprisingly, RNA blot analysis of leaf tissue harvested from 28
potentially transgenic P10 plant lines identified by PCR did not
sufficiently distinguish the plants to allow down-selection of
the lines for subsequent investigation. Moreover, though
Southern blotting showed that plant line P10-19 possesses at
least twice the transgene copy number of line P10-13, the two
lines expressed comparable P10 transcript levels in leaf.
Moderately higher leaf transcript levels detected in a few lines
did not correlate with higher levels of P10 protein in those
lines in later experiments. According to observed wound
inducibility of the super-promoter in a separate study,
conducted in our
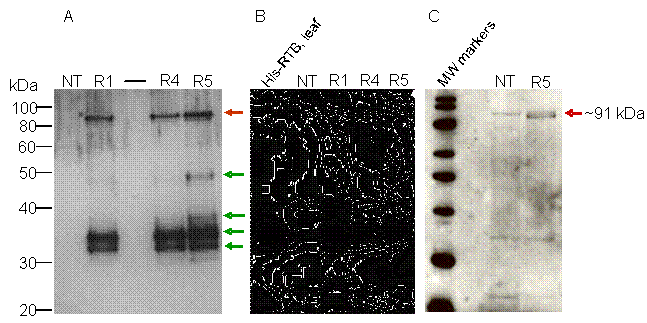
Figure 8. Western
blot analyses of lactose affinity-purified protein from P10
hairy root lines.
Extracts were prepared from 20 g FW of 12-14 day-old, P10-19
hairy root lines (R1, R4, R5), 10 g FW of hairy roots of
non-transformed tobacco (NT), and 5 g FW of leaves of the
positive control transgenic line, His:RTB (Reed et al., 2005)
and subjected to lactose affinity purification. For the P10 and
non-transformed hairy root samples, about 3 g FW equivalent of
hairy root samples were loaded per gel lane, while 0.5 g FW
equivalent of His:RTB leaf was loaded for Western blots using:
(A) rabbit polyclonal anti-RTB primary antibody at 1:5000
dilution, secondary goat anti-rabbit-HRP (Sigma, St. Louis, MO)
at 1:3000, with nitrocellulose membrane; (B) monoclonal
anti-His tag primary antibody at 1:1000 (Novagen, Madison, WI),
secondary goat anti-mouse-HRP (Sigma, St. Louis, MO) at 1:5000,
with nitrocellulose membrane; and (C) monoclonal anti-V
primary antibody at 1:100 dilution (BioDesign International,
Saco, ME), goat anti-mouse-HRP (Sigma, St. Louis, MO) at 1:5000,
with PVDF membrane. The red arrows denote the expected migration
position for full-length P10 protein, while the green arrows
denote likely P10 degradation products.
lab (Ñopo et al., manuscript submitted) we also attempted to elevate
P10 transcript expression levels by tissue wounding. However,
significantly or reproducibly higher levels of P10 mRNA in leaf
tissue of wounded plants compared to the non-wounded controls were
not obtained (data not shown).
We
expect from the current study and other work in our lab, as well as
a recent publication from the developer of the super-promoter (Lee
et al., 2007) that this promoter is developmentally regulated with
leaf age, such that small age differences in the leaf samples
harvested may partially explain the variable P10 transcript levels
across the three leaf Northern blot experiments reported herein.
Perhaps the failure of the transcript to
accumulate to higher levels in P10 plants reflects gene silencing of
the construct or inherent instability of the mRNA, despite our
previous success in expressing recombinant RTB constructs in tobacco
(Medina-Bolivar et al., 2003; Reed et al., 2005) and our current
efforts to eliminate RNA instability elements when designing the
synthetic F1 and V genes.
Tissue localization of P10 mRNA showed relatively low levels of
expression in all plant tissues, with expression in roots showing
the most promise for potential harvest of useful quantities of P10
protein. Therefore, we immediately initiated generation of hairy
roots from all available P10 plant lines with the expectation that
hairy roots may express the vaccine candidate at levels conducive to
protein purification and downstream assessments.
Immunoblot analysis showed that the predicted 91 kDa P10 protein was
purified from P10-19 R1, R4 and R5 hairy root lines. We speculate
that the cloning junction between RTB and F1 may have generated a
protease recognition site within the recombinant protein, resulting
in reduced recovery of full-length P10 protein coupled with recovery
during purification of the observed His-RTB-containing products in
the 35 kDa range. Similarly, our previous work with RTB-GFP
expressed in tobacco leaves and hairy roots showed that this
recombinant fusion protein was subject to degradation
(Medina-Bolivar et al., 2003). The banding patterns observed in the
35 kDa range in both the anti-RTB and anti-His blots recapitulate
our recently published data for the expression and lactose affinity
purification of His-RTB in tobacco (Reed et al., 2005). Cursory
attempts to stabilize P10 during extraction and purification by the
inclusion of protease inhibitors and chelating agents did not
increase the yield of recombinant protein.
Our
recovery of the fully-glycosylated 91 kDa form of P10 is significant
because we expect that RTB glycosylation may be important for its
function at the mucosal epithelium at the time of immune stimulation
by the antigen. Our successes with affinity purification and ELISA
demonstrate functionality of P10 as a lectin, including both
galactose- and lactose-binding capabilities known for RTB.
Additionally, our Western blot data show that three distinct domains
of the 91 kDa P10 molecule (V, RTB, and His portions) are probably
largely intact. This is an important consideration for accurate
presentation of our intended F1:V antigen.
We anticipate that
further optimization of the gene sequence may be required to
increase levels of P10 RNA. In addition, we expect increased yield
of purified P10 protein from hairy roots to be realized through
optimization of the extraction and purification processes. An
immunization trial utilizing purified P10 protein is being designed
to test the ability of the recombinant antigen to stimulate both
systemic and mucosal antibody responses in nasally immunized mice,
which will be considered the initial indicators of a “good”
response. As we aim for increased P10 yield from hairy roots, the
potential instability of the P10 mRNA and the potential inducibility
of the super-promoter system by various stimuli are some of the
targets to be addressed.
CONCLUSION
This report demonstrates
successful expression and purification of a functional, full-length,
glycosylated, His:RTB:F1:V protein fusion product from tobacco hairy
roots. This study also suggests that the hairy root system may be
especially well suited for expression of transgenes driven by the
super-promoter system, as supported in depth by another report from
our laboratory (Ñopo et al., manuscript submitted).
Assessment of the purified His:RTB:F1:V
recombinant protein’s immunomodulatory potential in vivo will
further address whether this novel antigen:adjuvant
combination may prove effective for induction of mucosal immunity
against pneumonic plague.
MATERIALS AND METHODS
Construction of the
binary vector (pP10) used for expression of recombinant
6His:Pat:RTB:F1:V vaccine in tobacco plants and hairy roots.
DNA oligomers encoding
the tobacco sequence-optimized F1 gene flanked by XhoI and
EcoRI restriction sites, and the sequence-optimized V gene
flanked by EcoRI and SacI restriction sites were
custom synthesized commercially (Blue Heron Biotechnology; Bothell,
WA). The F1 fragment was subcloned into pUC119 as an XhoI/EcoRI
fragment to create plasmid pUC119-F1. The V gene was subcloned into
pUC119 as a EcoRI/SacI fragment to create plasmid
pUC119-V. Plasmid pBC-RTB-F1-V was created by ligating the RTB
XbaI-XhoI fragment from pBC-R6-2 (Medina-Bolivar,
personal communication), the F1-containing XhoI/EcoRI
fragment from pUC119-F1, and the V-containing EcoRI/SacI
fragment from pUC119-V into the XbaI/SacI fragment of
pBC in a single reaction (Stratagene; La Jolla, CA). The KpnI/SacI
fragment of pBC- RTB-F1-V that contains the carboxyl-terminal
portion of the RTB gene plus F1:V was subcloned into pE1802
containing the super-promoter:TEV module (Ni et al., 1995) to create
the plasmid pP9. Plasmid pP9 was digested with KpnI and
treated with calf intestinal alkaline phosphatase prior to ligation
with the KpnI fragment from pBC-R9-His (Reed et al., 2005)
containing Pat (signal peptide from patatin), a 6XHis purification
tag, and the amino-terminal portion of RTB to create pP10.
Immediately downstream to the KpnI site and upstream of the
DNA sequence encoding the initial methionine of the patatin signal
peptide, an adenine was added (Figure 1). The ligation junction
within pP10 at the KpnI site internal to the RTB coding
sequence was sequenced to verify the predicted DNA sequence.
Plasmid pP10 was used to generate transgenic tobacco plants and
hairy roots in the current work.
Generation of
transgenic tobacco plants and hairy roots
Plasmid pP10 was
mobilized into Agrobacterium tumefaciens LBA4404 by the
freeze-thaw method (Holsters et al., 1978). A. tumefaciens
containing binary vector pP10 (Figure 1) was used to transform N.
tabacum cv. Xanthi according to a petiole-inoculation method
described previously (Medina-Bolivar and Cramer 2004). Transgenic T0
plantlets were micropropagated in PhytatrayTM II vessels
(Sigma, St. Louis, MO) containing mMS medium (Medina-Bolivar et al.,
2003), supplemented with 100 mg/L kanamycin. Cultures were
maintained under a 16 h photoperiod, at 24oC with average
light intensity of 98 μmol m-2 s-1. Hairy
roots were developed from the designated transgenic plant lines and
maintained as described before (Medina-Bolivar and Cramer, 2004).
Control plant material
Control plant materials
included plant line R6-2 (de35S:TEV:Pat:RTB:GFP, Medina-Bolivar et
al., 2003) and R9-His (de35S:TEV:Pat:RTB:His, Reed et al., 2005).
Genetic screening of
putative transgenic tobacco plants and hairy roots by PCR
Detection of RTB DNA in
putative transgenic plants was performed by PCR as follows. Genomic
DNA was isolated using DNeasy mini kit (Qiagen, Valencia, CA) and
subjected to PCR using RTB primers RB5
(5’-CGCTCTAGAGCTGATGTTTGTATGGAT-3’) and RB3a
(5’-GAGCTCCTCGAGAAATAATGGTAACCATAT-3’) with cycling parameters: 94°C
for 3 min; 35 cycles of 94°C
for 30 s, 50°C
for 30 s, 72°C
for 1 min 15 s; 72°C
for 10 min. The expected size of the PCR product is 800 bp.
Isolation of Genomic
DNA and Southern Blotting
Genomic DNA was isolated
from tobacco leaf and hairy root tissue using DNeasy Plant Maxi kit
(Qiagen, Valencia, CA). SacI-digested genomic DNA (10
μg
each sample) was resolved on a 0.7% agarose gel with DIG-labeled DNA
molecular weight markers (Roche, Boulder, CO). After
electrophoresis, the gel was stained with ethidium bromide and
photographed under UV illumination to document equal DNA loading per
well. The DNA was partially depurinated by incubation of the gel in
250 mM HCl for 20 min with shaking, followed by a brief rinse in
sterile water. DNA was denatured by incubation of the gel in 0.5 M
NaOH, 1.5 M NaCl with shaking, twice for 15 min each, followed by a
brief rinse in sterile water. The gel was neutralized by incubation
in 0.5 M Tris-HCl, 1.5 M NaCl, pH 7.5 with shaking, twice for 15 min
each. The gel was equilibrated in 20X saline sodium citrate (SSC)
buffer for 10 min and the DNA was transferred to positively-charged
nylon membrane (Roche, Boulder, CO) by capillary transfer overnight
in 20X SSC. Following transfer, the membrane was rinsed briefly in
2X SSC, and the DNA was cross-linked to the damp membrane by UV
illumination. Probe preparation and Southern blot hybridization and
detection are described below.
RNA Extraction and Northern Blot
Total RNA was extracted by the phenol/SDS method (Ausubel et al.,
2002) with modifications. Leaf or hairy root tissue (1 g) was
ground in liquid nitrogen and transferred to a tube containing 10 mL
RNA extraction buffer (0.18 M Tris, 0.09 M LiCl, 4.5 mM EDTA, 1% SDS,
pH 8.2) and 0.3 aqueous volume of TE-equilibrated phenol, pH 4.3.
Samples were vortexed vigorously for 1 min. Chloroform was added
(0.3 aqueous volume), followed by vortexing for 30 seconds. Samples
were centrifuged at 17,700 x g, 10 min, at 4°C to separate the
aqueous and organic phases. Phenol/chloroform extraction of the
aqueous layer was repeated twice, followed by two extractions with
chloroform alone. The aqueous layer was transferred to a fresh tube
on ice, and precipitated by addition of 0.1 volume 3 M NaOAc, pH
5.2, and 2.5 volumes 100% ethanol and incubation at –70°C for 30
minutes. Samples were centrifuged at 17,700 x g, 15 min at 4°C, and
pellets were resuspended in 1 mL DEPC-treated water. RNA was
selectively precipitated by addition of LiCl to a final
concentration of 2 M and incubation at 4°C overnight, followed by
centrifugation at 17,700 x g, 20 min, at 4°C. The RNA pellet was
washed with 1 mL of ice cold 2 M LiCl and resuspended in 100
μL
DEPC-treated water. For northern blots, 20
mg
of RNA per sample was processed as described before (Reed et al.,
2005) except that a digoxigenin (DIG)-labeled RTB probe was used, as
described below.
Preparation of DIG-labeled RTB probe
The
RTB probe was prepared by PCR from plasmid p10 as follows. The
reaction contained 1 ng of plasmid template, 0.5
mM
each RTB primer (RB5 and RB3a, described above), 0.2 mM each dATP,
dCTP, dGTP, 0.167 mM dTTP, 0.033 mM DIG-dUTP (Roche, Boulder, CO),
1.5 mM MgCl2, and 2.5 units Taq polymerase in a final
reaction volume of 100
mL.
PCR parameters were: denaturation at 94°C
for 3 min; 35 cycles of 94°C
for 30s, 50
°C
for 30s, 72°C
for 1 min 15 sec; 72°C
for 10 min.
Hybridization and detection of Northern and Southern blots
The
membrane was pre-hybridized in DIG Easy Hyb solution (Roche,
Boulder, CO), containing DIG Blocker (Roche, Boulder, CO) at 65°C
for 1 h. The probe was denatured by heating at 95°C
for 10 min and the blot was hybridized overnight at 65°C
in 2
mL
probe/mL DIG Easy Hyb solution containing DIG Blocker preheated to
65°C.
Following hybridization, the blot was washed twice for 20 min each
in 2X SSC, 0.1% SDS, and then twice for 20 min each in 0.5X SSC,
0.1% SDS, preheated to 65°C.
For chemiluminescent detection, the blot was processed according to
the manufacturer’s recommendations using the DIG Wash and Block
Buffer Set (Roche,Boulder, CO), and developed using
anti-DIG-alkaline phosphatase conjugate (Roche, Boulder, CO) and CDP-Star
substrate (Roche, Boulder, CO).
Protein Extraction
Tobacco hairy root tissue was harvested from culture, drained on
paper towels, and stored at -70°C until use. Protein extracts were
prepared as follows: hairy root tissue was ground under liquid
nitrogen with mortar and pestle and transferred to a centrifuge tube
containing Tris-ascorbate buffer [100 mM Tris base, 100 mM ascorbic
acid, 150 mM NaCl, 2.5% (w/v) PVP-40, 0.1% Tween 20; pH 7.0] at 3:1
buffer to tissue ratio and vortexed vigorously for 1 min. Extracts
were centrifuged at ~ 13,000 x g for 10 min at 4°C and supernatants
were filtered through four layers of MiraclothTM (Calbiochem-EMD
Biosciences, San Diego, CA). The total protein concentration of
crude protein extracts was measured using the Advanced Protein Assay
(Cytoskeleton, Denver, CO) in a Bio-Tek EL808 Ultra Microplate
Reader. Total protein determinations were made in reference to
bovine albumin serum (BSA) standards dissolved in Tris-ascorbate
buffer.
Asialofetuin-binding ELISA
The
functionality of recombinant protein in the protein extracts of
transgenic lines was determined via binding to asialofetuin, the
glycoprotein fetuin treated with sialydase to expose galactose-terminated
glycans as we described before (Reed et al., 2005). Two hundred
microliters of asialofetuin (Sigma; St. Louis, MO) at a
concentration of 300
μg/mL
in modified PBS (mPBS) buffer (100 mM Na-phosphate, 150 mM NaCl, pH
7.0) was bound per well of an Immulon 4HBX (Fisher, Pittsburg, PA)
microtiter plate for 1 h at room temperature (RT). The coating
solution was discarded, and the wells were blocked with 200
μL
of 3% BSA, 0.1% Tween 20 in PBS for 1 h at RT. The blocking
solution was discarded and 100
μL
each of RTB standards (described below) and sample protein extracts
(prepared as above) was applied in triplicate wells and incubated
for 1 h at RT. The blocking solution was discarded, and wells were
washed three times with PBS, 0.1% Tween 20. Rabbit anti-R.
communis lectin (RCA60) polyclonal antibody (Sigma, St. Louis,
MO) diluted at 1:4000 in blocking buffer (as above) was applied and
incubated for 1 h at RT, then discarded. The wells were then washed
as before. Alkaline phosphatase-conjugated goat-anti-rabbit IgG
(Bio-Rad; Hercules, CA) was applied at a 1:3000 dilution in blocking
buffer and incubated for 1 h at RT, then discarded. The wells were
washed three times as before, and 100
μL
pNPP (p-nitrophenyl phosphate disodium salt) substrate (Pierce,
Rockford, IL) was applied per well. The reaction was stopped after
15 min by the addition of 50
μL
of 2 N NaOH. Absorbance (A405nm) was read in a Bio-Tek
EL808 Ultra Microplate Reader. One hundred µg of total protein
extract from leaves or hairy root tissue or 100
mL
of media from hairy root cultures were assessed compared to a
standard curve consisting of serially diluted castor bean-derived
RTB (Vector Labs, Burlingame, CA) in Tris-acorbate buffer (above),
in concentrations ranging from 2.5 ng to 500 ng RTB per well, and
were plotted as 1/A405nm vs. 1/ng RTB per well.
Lactose affinity
chromatography
Recombinant protein was
purified from hairy root tissue by carbohydrate-affinity
chromatography using lactose-conjugated agarose resin (EY Labs, San
Mateo, CA). A 500-μL
aliquot of resin was added to clarified crude protein extracts
prepared as above from 10 g FW hairy root tissue in 50-mL conical
tubes and incubated with continuous immersion on a rotary Labquake
shaker (Barnstead, IA) for 1-2 h at 4°C and washed three times for 5
min in 5 mL ice-cold PBS buffer (Sambrook et al., 1989), pH 7.4. A
final wash was conducted in 0.5 mL volume, during which the resin
was transferred to a 1.5 mL microfuge tube for elution. Bound
protein was eluted with 0.5 ml ice-cold elution buffer (0.5 M
galactose in PBS, pH 7.4). Eluted fractions were concentrated
approximately 10-fold in Centricon-10 units (Millipore, Bedford, MA)
at 4°C according to the manufacturer’s instructions and stored at
-20°C for subsequent analyses.
Western blotting
Protein samples were
resolved by SDS-PAGE NuPAGETM 10% Bis-Tris acrylamide
gels in an X-Cell SureLockTM Mini-Cell apparatus (Invitrogen,
Carlsbad, CA) according to manufacturer’s recommendations using MOPs
running buffer. After electrophoresis for 60 min at 200 volts,
protein was transferred to 0.2 µm Trans-Blot® nitrocellulose
membrane (Bio-Rad, Hercules, CA) or PVDF as indicated using an
X-Cell IITM blot module and NuPAGE transfer buffer
containing 20% MeOH for 1.5 h at 30 V. Blots were blocked overnight
shaking at 4°C in blocking solution (PBS containing 0.1% Tween-20,
3% BSA) followed by incubation with the designated primary antibody
at the designated concentration in blocking solution for 1 h.
Membranes were washed three times for 15 min each in PBS,
0.1% Tween-20 and incubated with secondary antibody as indicated for
45 min to 1 h and washed 3 times as above. Chemiluminescent
detection was performed using Immun-StarTM AP substrate
(Bio-Rad; Hercules, CA) and Nitro-Block Enhancer IITM (Tropix,
Bedford, MA).
Statistical analysis
All statistics were
determined by ANOVA (Dunnett’s t Test to compare P10 protein
expression against a control, with
α
= 0.05), with the aid of the Statistical Analysis Systems
statistical software, release 8.00.
Acknowledgements
The authors gratefully
acknowledge the financial support of NIH through the STTR grant to
Biodefense Technologies Inc., Blacksburg, Virginia, the State of
Virginia Commonwealth Technology Research Fund and the Arkansas
Biosciences Institute at Arkansas State University.
LITERATURE CITED
Alpar, H.O., J.E. Eyles,
E.D. Williamson, S. Somavarapu. (2001) Intranasal vaccination
against plague, tetanus, and diphtheria. Adv. Drug Deliv. Rev.
51:173-201.
Alvarez, M.L., H.L.
Pinyerd, J.D. Crisantes, M.M. Rigano, J. Pinkhasov, A.M. Walmsley,
H.S. Mason, G.A. Cardineau. (2006) Plant-made subunit vaccine
against pneumonic and bubonic plague is orally immunogenic in mice.
Vaccine 24:2477-2490.
Anderson, G.W., Jr., D.G.
Heath, C.R. Bolt, S.L. Welkos, A.M. Friedlander. (1998) Short- and
long-term efficacy of single-dose subunit vaccines against
Yersinia pestis in mice. Am. J. Trop. Med. Hyg. 58:793-799.
Ausubel A., R. Brent, R.
Kingston, D. Moore, J. Smith, K. Struhl. (2002) Current Protocols in
Molecular Biology. John Wiley & Sons Inc., New York
Bertherat, E., K.M.
Lamine, P. Formenty, P. Thuier, V. Mondonge, A. Mitifu, L. Rahalison.
(2005) Major pulmonary outbreak in a mining camp in the Democratic
Republic of Congo: brutal awakening of an old scourge. Med. Trop.
(Mars) 65:511-514.
Cohen, R.J., J.L.
Stockard. (1967) Pneumonic plague in an untreated plague-vaccinated
individual. JAMA 202:365-366.
Du, Y., R. Rosqvist, A.
Forsberg. (2002) Role of fraction 1 antigen of Yersinia pestis
in inhibition of phagocytosis. Infect. Immun. 70:1453-1460.
Fields, K.A., M.L. Nilles,
C. Cowen, S.C. Straley. (1999) Virulence role of V antigen of
Yersinia pestis at the bacterial surface.
Infect. Immun.
67:5395-5408.
Galimand, M.,
A. Guiyoule, G.Gerbaud, B. Rasoamanana, S. Chanteau, E. Carniel, P.
Courvalin. (1997)
Multidrug resistance in Yersinia pestis mediated by a
transferable plasmid.
N. Engl. J.
Med. 337:677-680.
Guiyoule, A.,
G. Gerbaud, C. Buchreiser, M. Galimand, L. Rahalison, S. Chanteau,
P. Courvalin, E. Carniel.
(2001) Transferable
plasmid-mediated resistance to streptomycin in a clinical isolate of
Yersinia pestis. Emerg. Infect. Dis. 7:43-48.
Glynn, A., L.C. Freytag,
J.D. Clements. (2005) Effect of homologous and heterologous
prime-boost on the immune response to recombinant plague antigens.
Vaccine 23:1957-1965.
Heath, D.G., G.W.
Anderson, Jr., J.M. Mauro, S.L. Welkos, G.P. Andrews, J. Adamovicz,
A.M. Friedlander. (1998) Protection against experimental bubonic and
pneumonic plague by a recombinant capsular F1-V antigen fusion
protein vaccine. Vaccine 16:1131-1137.
Holsters M., D. de Waele,
A. Depicker, E. Messe, M. van Montagu, J. Schell. (1978)
Transfection and transformation of Agrobacterium tumefaciens.
Mol. Gen. Genet. 163:181-187.
Jones, S.M., F. Day, A.J.
Stagg, E.D. Williamson. (2000) Protection conferred by a fully
recombinant sub-unit vaccine against Yersinia pestis in male
and female mice of four inbred strains. Vaccine 19:358-366.
Jones, S.M., K.F.
Griffin, I. Hodgson, E.D. Williamson. (2003) Protective efficacy of
a fully recombinant plague vaccine in the guinea pig. Vaccine
21:3912-3918.
Jones, T., J.J. Adamovicz,
S.L. Cyr, C.R. Bolt, N. Bellerose, L.M. Pitt, G.H. Lowell, D.S.
Burt. (2006) Intranasal Protollin/F1-V vaccine elicits respiratory
and serum antibody responses and protects mice against lethal
aerosolized plague infection. Vaccine 24:1625-1632.
Lee, L.Y., M.E. Kononov,
B. Bassuner, B.B. Frame, K. Wang, S.B. Gelvin. (2007) Novel plant
transformation vectors containing the Super-promoter. Plant
Physiol. 145:1294-1300.
Medina-Bolivar, F., R.
Wright, V. Funk, D. Sentz, L. Barroso, T.D. Wilkins, W. Petri, Jr.,
C.L. Cramer. (2003) A non-toxic lectin for antigen delivery of
plant-based mucosal vaccines. Vaccine 21:997-1005.
Medina-Bolivar, F., C.L.
Cramer. (2004) Production of recombinant proteins by hairy roots
cultured in plastic sleeve bioreactors. Methods Mol. Biol.
267:351-363.
Mett, V., J. Lyons, K.
Musiychuk, J.A. Chichester, T. Brasil, R. Couch, R. Sherwood, G.A.
Palmer, S.J. Streatfield, V. Yusibov. (2007) A plant-produced plague
vaccine candidate confers protection to monkeys. Vaccine
25:3014-3017.
Meyer K.F. (1970)
Effectiveness of live or killed plague vaccines in man. Bull. World
Health Organ. 42:653-666.
Mudur, G. (1995) India's
pneumonic plague outbreak continues to baffle.
BMJ
.311:706.
Ni, M., D.
Cui, J. Einstein. S. Narasimhulu, C.E. Vergara, S.B. Gelvin.
(1995) Strength and
tissue specificity of chimeric promoters derived from the octopine
and mannopine synthase genes. Plant J. 7:661-676.
Reed, D.G., L.H. Ñopo, V.
Funk, B.J. Woffenden, M.J. Reidy, M.C. Dolan, C.L. Cramer, F.
Medina-Bolivar. (2005) Expression of functional hexahistidine-tagged
ricin B in tobacco. Plant Cell Rep. 24:15–24.
SAS (1999) Statistical
Analysis System, release 8.00. Cary, NC. Statistical Analysis System
Institute, Inc.
Titball, R.W., A.M.
Howells, P.C.F. Oyston, E.D. Williamson. (1997) Expression
of the Yersinia pestis capsular antigen (F1 antigen) on the
surface of an aro A mutant of Salmonella typhimurium induces
high level protection against plague. Inf. Immun. 65: 1926-1930.
Williamson,
E.D., S.M. Eley, A.J. Stagg, M. Green, P. Russell. R.W. Titball,
R.W. (1997) A subunit vaccine elicits IgG in serum, spleen cell
cultures and bronchial washings and protects immunized animals
against pneumonic plague. Vaccine 15:1079-1084.
Williamson,
E.D., S.M. Eley, A.J. Stagg, M. Green, P. Russell, R.W. Titball.
(2001) A single dose subunit vaccine protects against pneumonic
plague. Vaccine 19:566-571.
Williamson,
E.D., H.C. Flick-Smith, C. Le Butt, C.A. Rowland, S.M.Jones, E.L.
Waters, R.J. Gwyther, J. Miller, P.J. Packer, M. Irving. (2005)
Human immune response to a plague vaccine comprising recombinant F1
and V antigens. Infect. Immun. 73:3598-3608.
Williamson,
E.D., H.C. Flick-Smith, E. Waters, J. Miller, I. Hodgson, C.S. Le
Butt, J. Hill. (2007) Immunogenicity of the rF1+rV vaccine for
plague with identification of potential immune correlates.
Microb. Pathog. 42:11-21.
Accepted for
publication: 7 October 2008
|